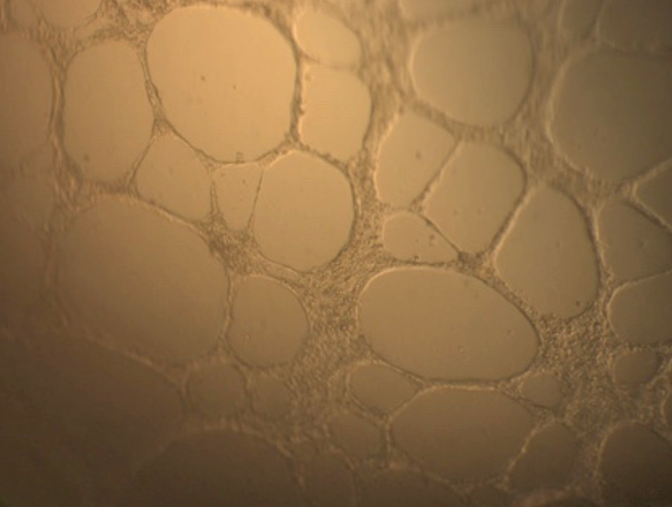
Conditioned media from human adipose-derived stem cell culture in some stressed culture conditions differ angiogenic potential
- Stem Cell Institute, University of Science Ho Chi Minh City, Viet Nam
- Viet Nam National University Ho Chi Minh City, Viet Nam
Abstract
Introduction: The potency of mesenchymal stem cells (MSCs) related to their biological effects includes immune modulation and angiogenesis. Recently, some stress conditions were applied to enhance the angiogenic potential of MSCs. This study aimed to assess the effects of conditioned media (CM) collected from adipose-derived stem cells’ (ADSCs’) culture under various stress conditions on angiogenesis in vitro.
Methods: ADSCs were isolated and expanded according to a published protocol. These cells were treated with some stress conditions including hypoxia, starvation, a combination of hypoxia and starvation, and TNF-alpha treatment. CM from these cultures were collected and used for further experiments. The angiogenic potential of CM was evaluated through stimulation of HUVECs to form vessels in vitro. ELISA was used to measure the VEGF concentrations in CM.
Results: CM-derived various stress ADSC cultures differently affected angiogenesis of HUVECs. The supernatant from a hypoxic culture of ADSCs contained the highest concentration of VEGF and was higher than normoxic culture. However, in others, VEGF concentrations in CM significantly reduced compared to control. CM from TNF-alpha treatment failed to support the formation of blood vessels from HUVECs, while other conditions could support blood vessel formation in vitro. TNF-alpha dually affected both ADSCs and HUVECs. Furthermore, TNF-alpha could stimulate or suppress the VEGF production in dose-response in ADSCs and cause apoptosis in HUVECs at high concentrations.
Conclusion: CM from the hypoxic culture of ADSCs contained a high concentration of VEGF, supporting angiogenesis of HUVECs well. This is a simple technique that can be used in translational applications. However, the use of TNF-alpha yielded dual effects on ADSCs and HUVECs. Although the VEGF production was enhanced at a low dose of TNF-alpha, they could induce apoptosis in endothelial cells to cause the failure of angiogenesis.
Introduction
Mesenchymal stem cells (MSCs) are the most commonly used stem cells in clinical applications. The potency of MSCs is generally based on their immune modulation and angiogenesis that can promote tissue regeneration and inhibit scarring1, 2, 3. Some recent studies have shown that the biological effects of MSCs depend on their secretomes4, 5, 6, 7. Moreover, MSC-derived secretomes can cause bioeffects compared to whole living cells8, 9.
Ho . (2017) showed that both MSCs and their conditioned media (CM) could enhance uterine defect repair in vivo through paracrine involvement IL-68. CM-MSCs could also reduce cartilage damage and modulate the immune responses in inflammatory arthritis in mice5, ameliorate Parkinson’s symptoms in rat models9, protect cholinergic neurons in the medial septum10, and prevent the ovarian injury through cisplatin in animal models11. In animal models, Hong . (2020) showed that CM-MSCs could decrease the apoptosis of oocytes and granulosa cells affected by cisplatin11.
Some studies have demonstrated the role of CM-MSCs in angiogenesis12, 13. Shen . (2015) demonstrated that CM from umbilical-cord-derived MSCs could enhance angiogenesis through SDF-1, MCP-1, HGF, VCAM-1, IL-8, IGF-1, and VEGF12. Lu (2018) proved that CM from adipose-derived stem cells (ADSCs) were more efficient in angiogenesis than MSCs from the umbilical cord and endothelium tissues13 in both and conditions. This characteristic depends on the higher levels of many cytokines, and MMPs produced in ADSCs than others, including VEGF, bFGF, PDGF-BB, IGN-gamma, IL-10, chemerin, MMP-9, and MMP-1313.
To improve the angiogenic effects of CM from MSC culture, there have been some recent efforts to treat MSCs in some stress conditions or gene modification14, 15, 16, 17, 18, 19. Ogisu . (2020) cultured MSCs from the bone marrow in cyclic stretch stimulation conditions for 24 h, and showed that their CM contained more VEGF, BMP-2, and BMP-4 than the control14. In anassay, the CM from the stress condition showed greater angiogenic effects than control14. Chen . (2014) demonstrated that CM from the hypoxia culture of MSCs enhanced wound healing20. CM from hypoxia culture of ADSCs also promoted the healing of the gastric mucosal injury in a rodent model21. In another study, Almeria . (2019) demonstrated that extracellular vesicles (EVs) from the hypoxic culture of MSCs significantly increased vascular tube formation in vitro in HUVECs compared to normoxia15. Furthermore, Zhu (2018) reported that exosomes derived from hypoxia CM of MSCs facilitated ischemic cardiac repair by ameliorating cardiomyocyte apoptosis16.
Starvation is another stress condition used to promote stemness and angiogenesis in MSCs as reported in a few articles17, 22, 23, 24. In a reduced serum condition (2% serum compared to the usual 10% serum), ADSCs reduced their proliferation but enhanced VEGF production23. However, a later study showed that MSCs cultured in a serum-reduced medium decreased the expression of VEGF, MCP-1, MIP-1 alpha, MIP-1 beta, MMP-2 compared to the normal level of serum22. Conversely, MSCs improved their exosome’s activities in a condition of serum deprivation24. In a recent study, Shin . (2019) showed that in the case of Wharton’s jelly-derived MSCs, they produced less protein in the serum-free medium than in the serum medium17.
Jin (2020) expressed FGF-2 in gingival MSCs (GMSCs) to produce MSCs with high expression of FGF-2 (FGFGMSCs)18. FGFGMSCs could produce more FGF-2, VEGF, and TGF-beta than the original GMSCs. The CM collected from the culture of FGFGMSCs could trigger the expression of PLGF, SCF, and VEGFR2 in HUVECs18. Similar to this strategy, Park. (2020) engineered MSCs to express HGF (HGFMSCs) and used them to repair cardiac injury in animal models. HGFMSCs could survive longer than the original cells (MSCs) in the 3D cardiac patch and conferred cardioprotection19.
In this study, we aimed to compare the angiogenic potential of CM derived from ADSC cultures in certain stress culture conditions including hypoxia (2% oxygen), starvation (0% serum), a combination of hypoxia and starvation, and a TNF-alpha treatment. Furthermore, we aimed to determine the suitable stress that can be used to produce CM from ADSCs with the strongest angiogenic potential from the results.
Methods
ADSC isolation and characterization
ADSCs were isolated and expanded as per the previous published works25, 26, 27. Adipose tissues were collected at the hospital with consent forms. These tissues were discarded during the aesthetic surgery. The use of these samples for the study was approved by the institutional ethical committee. At the laboratory, the tissues were washed twice with a washing buffer (PBS), and then the stromal vascular fraction (SVF) was extracted from the adipose tissue using the Cell Extraction Kit (Regenmedlab, HCMC, VN). The SVF pellet was re-suspended in the ADSCCult I primary (Regenmedlab, HCMC, VN) at the ratio of 10 SVF cells per mL. The cell suspension was plated in the T75 flask for five days without any replacement of the medium. After five days of incubation at 37 ℃ with 5% CO, the flasks were refreshed with 10 mL of fresh media. When the primary cells reached 70–80% confluency, they were sub-cultured in new flasks at a splitting ratio of 1:3 using the ADSCCult I medium (Regenmedlab, HCMC, VN). The ADSCs in the 5passages were characterized as MSC phenotypes as suggested by ISCT for minimal MSC criteria28.
ADSCs checked the surface marker expression by flow cytometry. The expression of CD14, CD34, CD44, CD45, CD73, CD90, CD105, and HLA-DR was evaluated using the respective antibodies. Briefly, for each marker, the 5.10 cells were resuspended in 100 mL of staining buffer (BD Bioscience, MA), and then 5 uL of monoclonal antibody conjugated with dye were added into the tube an incubated at RT for 30 min in the dark. Thereafter, the tubes were centrifuged to remove the extra-monoclonal antibody and washed twice using the washing buffer. The Stained cells were analyzed by FACSCalibur flow cytometry and FlowJo software (both from BD Bioscience, MA).
The differentiation potential of ADSCs was checked as per the previous published works [25-27]. ADSCs were cultured and induced in commercial differentiation media including adipogenesis, osteogenesis, and chondrogenesis to enable their differentiation into adipocytes, osteoblasts, and chondroblasts, respectively (Thermo Fisher, MA). On the 21 day after differentiation, the induced cells were stained with Oil Red O, Alizarin Red, and Safranin O to confirm the phenotypes of adipocytes, osteoblasts, and chondrocytes, respectively.
Conditioned media (CM) preparation
One million ADSCs were seeded in the T75 flask and incubated at 37 ℃ with 5% CO until reaching 70% confluency. The flasks were refreshed with fresh culture media. For group control (G0), hypoxia (group 3–G3) culture media (ADSCCult I completed medium, Regenemedlab) were used. For group 1 (G1), the completed culture medium with 100 ng/mL of TNF-alpha was used. For group 2 (G2) and group 4 (G4), the basal medium of ADSCCult I (DMEM/F-12) was used. The flasks of G3 and G4 were placed in the 5% oxygen incubators (Binder, Germany). The flasks of other groups were incubated in 20% oxygen, with 5% CO, and at 37 ℃. All flasks were incubated for 48 h; then, the supernatants were collected in 50 mL centrifuge tubes. The supernatants were centrifuged at 3,000 g at 4 ℃ for 15 min to remove debris. Finally, the CM were kept in a –86-℃ freezer for further experiments.
Blood vessel formation assay
This assay was performed using the Angiogenesis Starter Kit (Thermo Fisher, MA). First, the HUVECs included in the kit were expanded using the M200 medium supplemented with LVES (included in the kit). Subsequently, the Geltrex matrix was prepared as instructed and used to cover the surface of wells of 96-well plates. Thereafter, HUVECs were collected and re-suspended in CM (G0: control, G1: TNF-alpha, G2: starvation, G3: hypoxia 5% oxygen, and G4: hypoxia 5% oxygen and starvation) and positive control using M200 medium supplemented with LVES. Next, HUVECs were cultured in CM and M200 medium supplemented with LVES for 48 h at 37 ℃, with 5% CO, and in 20% oxygen. Finally, blood vessel formation was observed under an inverted microscope (Carl Zeiss, Germany).
VEGF concentration measurement
CM were collected and kept at –86 ℃ after removing the cell debris prior to use in this assay. The VEGF concentrations were measured using the VEGF ELISA kits (Abcam). All protocols were performed following the manufacturer’s guidelines.
Effects of TNF-alpha on VEGF secretion of ADSCs and apoptosis of HUVECs
A serial concentration of TNF-alpha (0, 10, 20, 40, 60, 80, and 100 ng/mL of TNF-alpha) was used to evaluate the effects of TNF-alpha on VEGF secretion of ADSCs. ADSCs were cultured in the 6-well plates with 10 cells per well in the culture medium (ADSCCult I completed medium, Regenmedlab, HCMC, VN) until 70% confluency. The media were removed, and the fresh media supplemented to 0, 10, 20, 40, 60, 80, and 100 ng/mL of TNF-alpha (Thermo Fisher, MA) were used with 2 mL per well. The cells were cultured in an incubator with 5% CO, at 37 ℃, and in 20% oxygen for 48 h. The CM were collected, and the cell debris was removed by centrifugation at 3,000 g at 4 ℃ for 15 min. These CM were then used to measure VEGF concentration using an ELISA kit (Abcam).
A serial concentration of TNF-alpha (0, 10, 20, 40, 60, 80, and 100 ng/mL of TNF-alpha) was used to evaluate the effects of TNF-alpha on the apoptosis of HUVECs. First, the HUVECs were seeded on the 6-well plates with 10 cells per well in the culture medium (M200 medium supplemented with LVES, Thermo Fisher) until 70% confluency. The media were removed, and the fresh media M200 (with LVES) supplemented to 0, 10, 20, 40, 60, 80, and 100 ng/mL of TNF-alpha (Thermo Fisher, MA) were used with 2 mL per well. The cells were cultured in an incubator with 5% CO, at 37 ℃, and in 20% oxygen for 48 h. The cells were then collected using the detachment reagent (Regenmedlab, HCMC, VN) and then evaluated for the percentage of apoptotic cells using the apoptosis kit, Annexin V/PI (BD Bioscience, MA). All assays were triplicated.
Statistical analysis
All assays were triplicated. Data were analyzed using the GraphPad Prism 8 software. The statistical analysis was performed using t-test. P-value < 0.05 was considered significant. All data were presented as mean ± standard deviation.
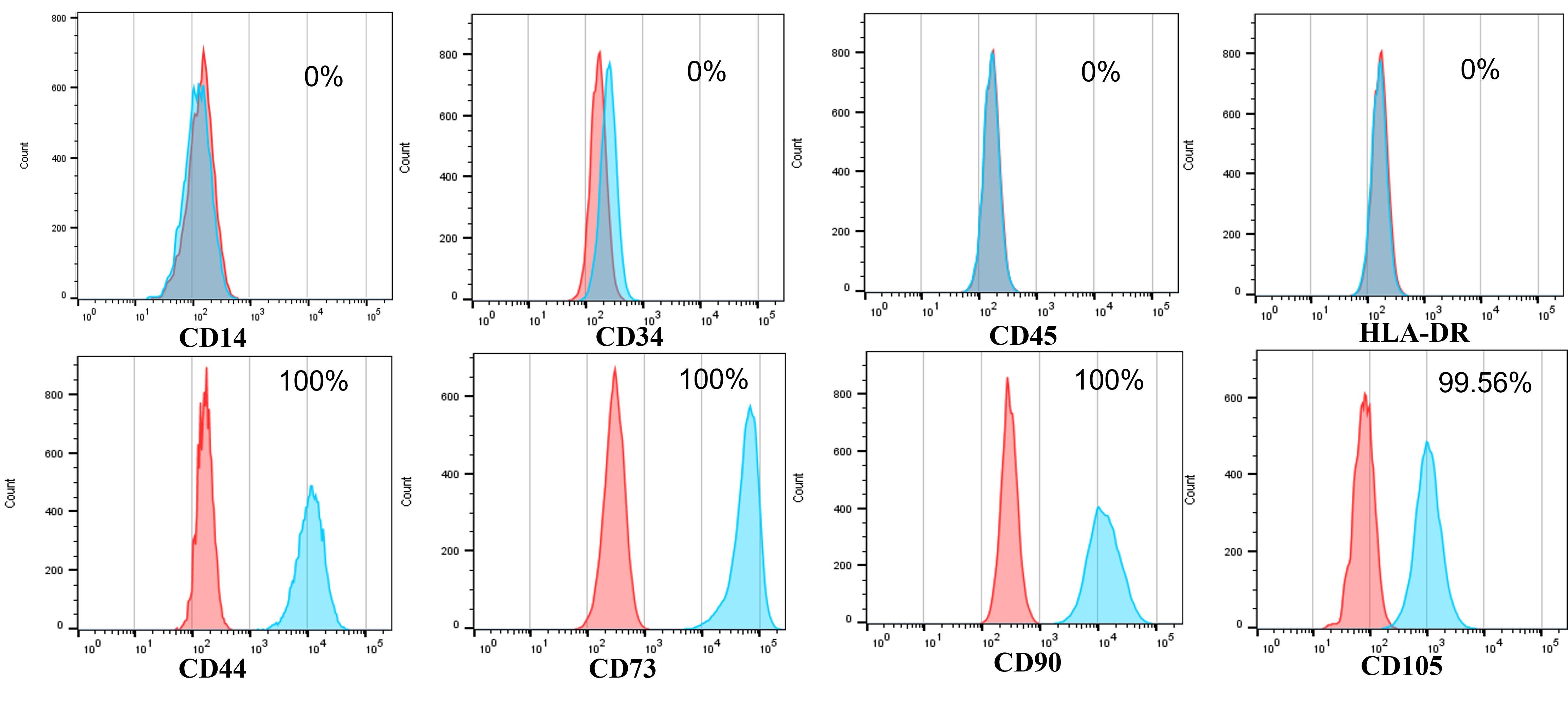
The isolated ADSCs from human adipose tissues displayed the particular marker profiles of MSCs. They highly expressed CD44, CD73, CD90, and CD105 and did not express CD14, CD34, CD45, and HLA-DR.
Results
Isolation and characterization of human ADSCs
The isolated ADSCs exhibited full characteristics of MSCs suggested by ISCT regarding minimal criteria of MSCs. They displayed the fibroblast-like shape when adhered to the surface of plastic flasks. They also revealed that the marker profiles of MSCs were positive with CD44 (100%), CD73 (100%), CD90 (100%), and CD105 (98.56%) and negative with CD14 (0 %), CD34 (0 %), CD45 (0 %), and HLA-DR (0 %) (Figure 1). These cells were successfully induced to osteoblasts that could be positive with Alizarin Red staining (Figure 2), chondroblasts that could be positive with Alcian blue staining (Figure 2), and adipocytes that could be positive with Oil Red O staining (Figure 2).
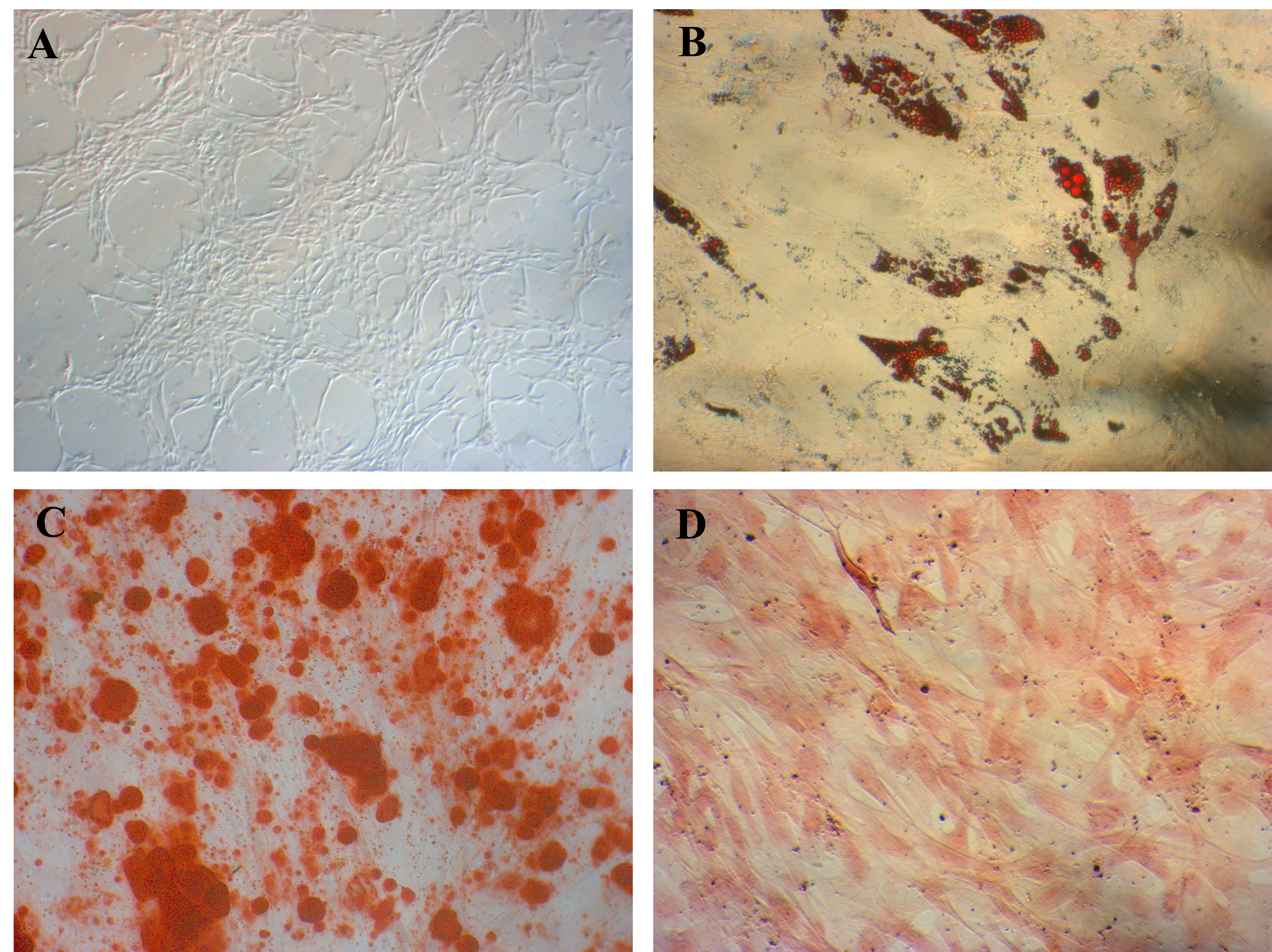
ADSC candidates could be successfully induced to adipocytes, osteoblasts, and chondrocytes in inducible media. After 21 days of induction, these cells could be positive to staining with Oil Red O (for adipogenesis induction), Alizarin Red (for osteogenesis induction), and Safranin O (for chondrogenesis induction).
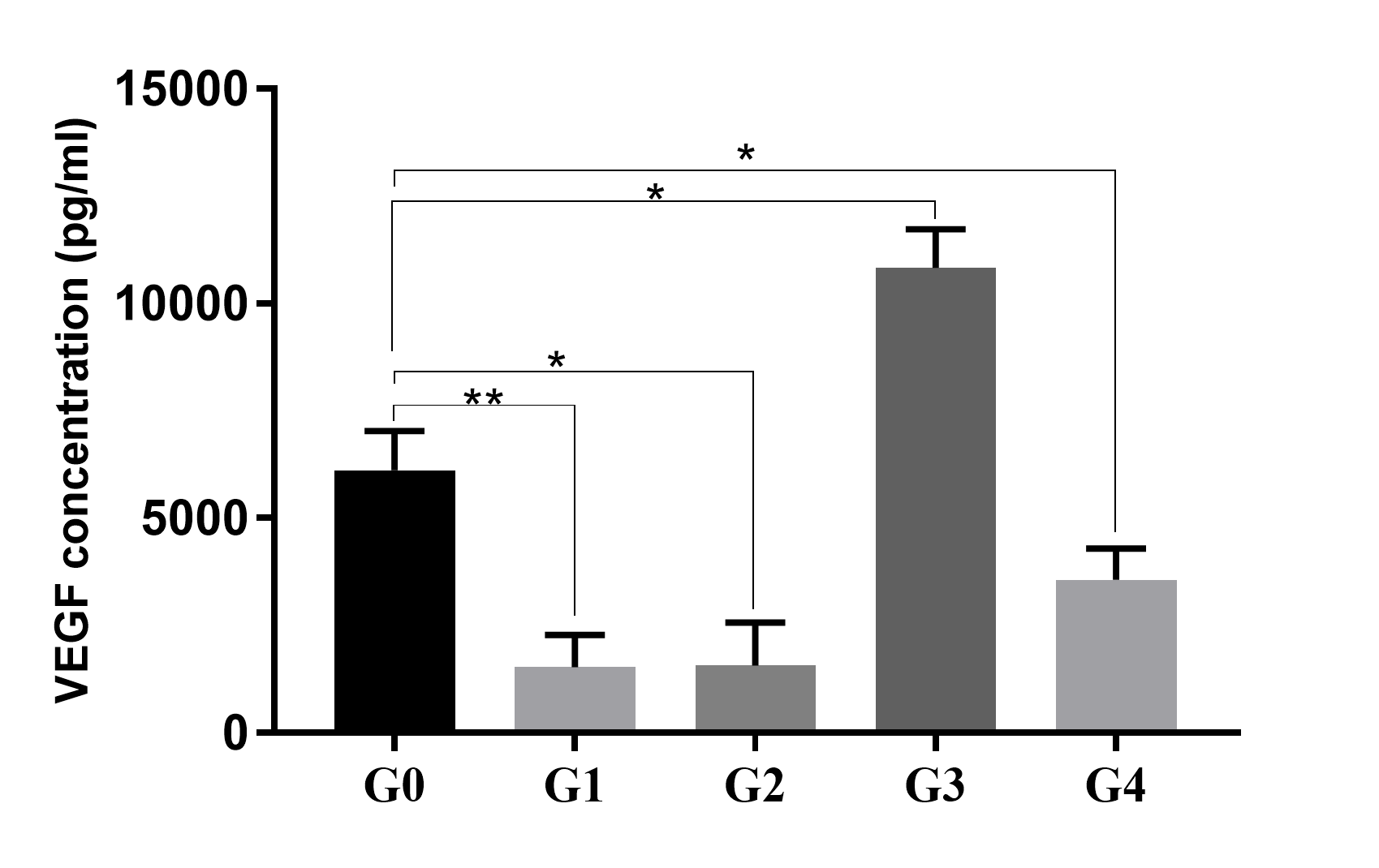
VEGF secretion in ADSC culture supernatants. In a normal culture condition (G0), ADSCs could secrete a high concentration of VEGF in the supernatant after 48 h of culture. The VEGF secretion significantly increased in hypoxia (G3) and significantly reduced in TNF-alpha treatment (G1), starvation (G2), or in a combination of hypoxia and starvation (G4). *p < 0.05 and **p < 0.005).
VEGF secretion under different conditions
The CM collected from different groups indicated different concentrations of VEGF. The highest concentration of VEGF was recorded in G3 in which the cells were treated in a hypoxia condition, and the lowest concentration of VEGF was recorded in G2 in which the cells were treated in TNF-alpha stress. The VEGF concentrations measured were 6,116 ± 527.9 pg/mL, 1,536 ± 432.1 pg/mL, 1,569 ± 710 pg/mL, 10,829 ± 640 pg/mL, and 3,574 ± 505 pg/mL in the supernatants of G0, G1, G2, G3, and G4, respectively (Figure 3).
The results revealed that in a normal culture condition (G0, control), ADSCs could produce a high concentration of VEGF in the CM; however, this concentration could be increased in a hypoxia condition (6,116 ± 527.9 pg/mL . 10,829 ± 640 pg/mL, respectively, for normoxia hypoxia condition; p <0.05). In the conditions of TNF-alpha treatment (G1), starvation (G2), or a combination of hypoxia and starvation (G4), the concentrations of VEGF in CM significantly reduced compared to the control (G0; p < 0.05). In conditions of TNF-alpha treatment (G1) and starvation (G2), the concentrations of VEGF were too low with a non-significant difference between these groups (1,536 ± 432.1 pg/mL . 1,569 ± 710 pg/mL, respectively, for G1 . G2; p > 0.05).
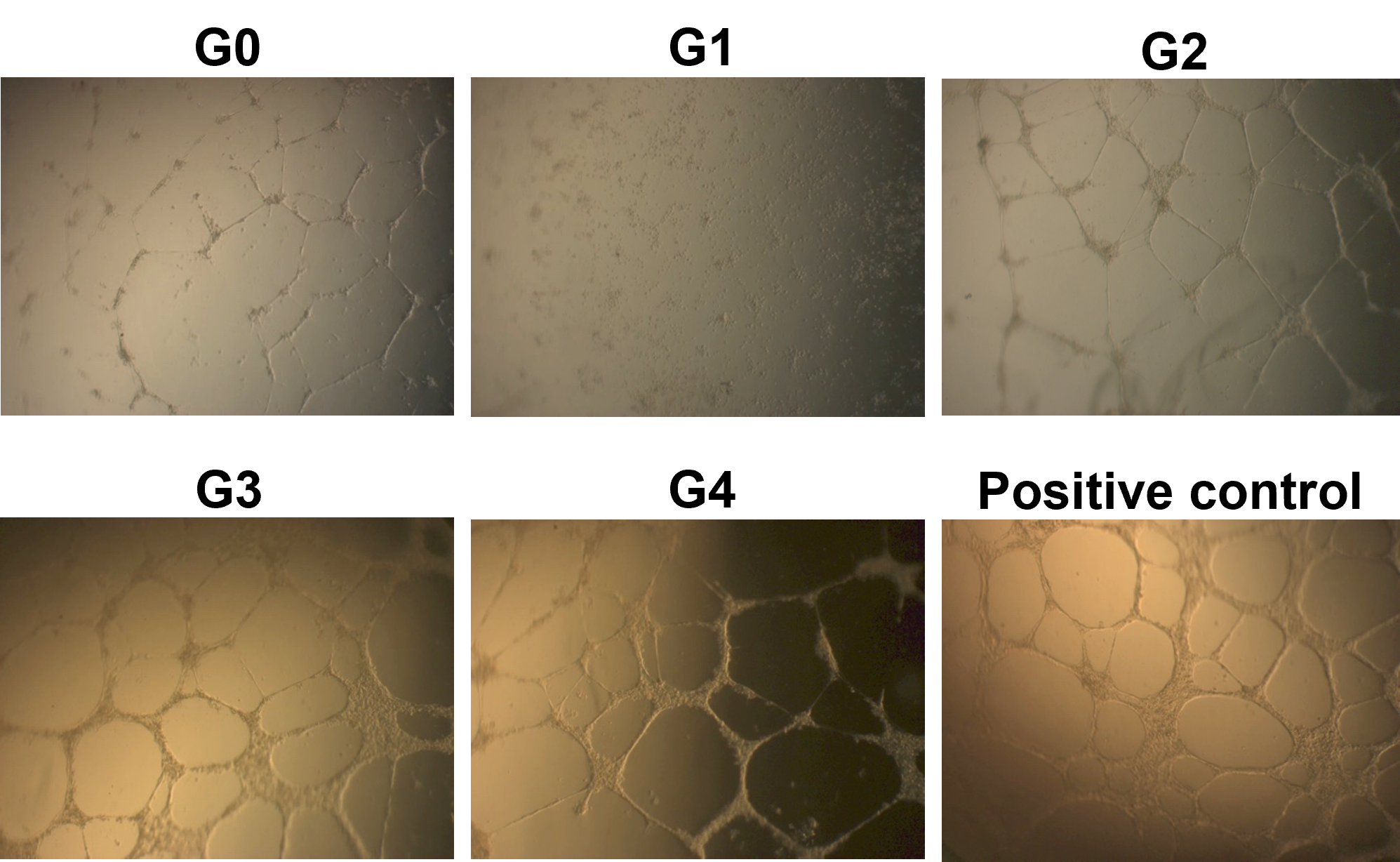
Blood vessel formation from HUVECs
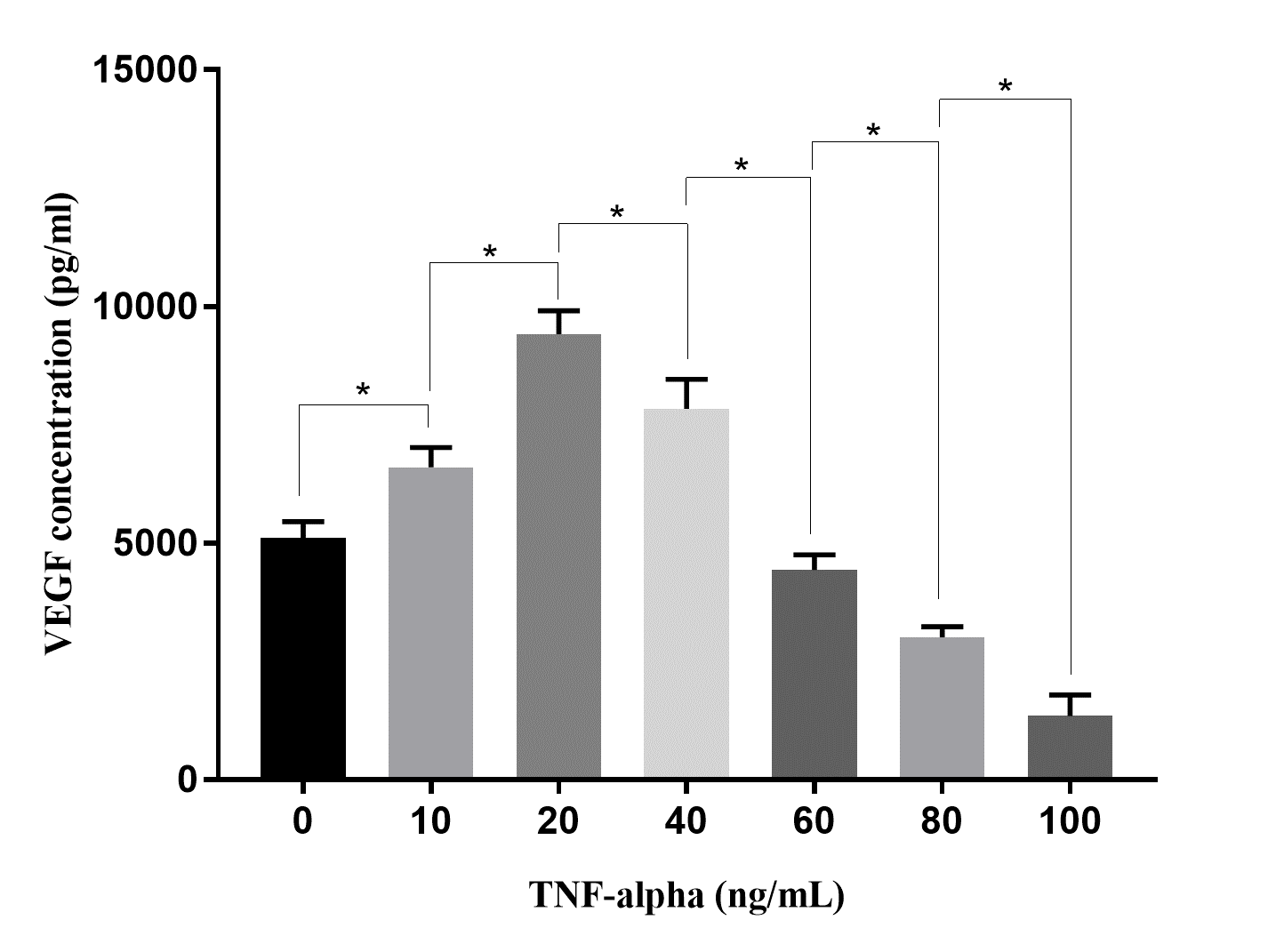
The VEGF secretion of ADSCs depends on the concentrations of TNF-alpha. At a low dose of TNF-alpha, TNF-alpha stimulated VEGF secretion, while at the high dose (from 40 ng/mL), TNF-alpha reduced VEGF secretion in ADSCs.
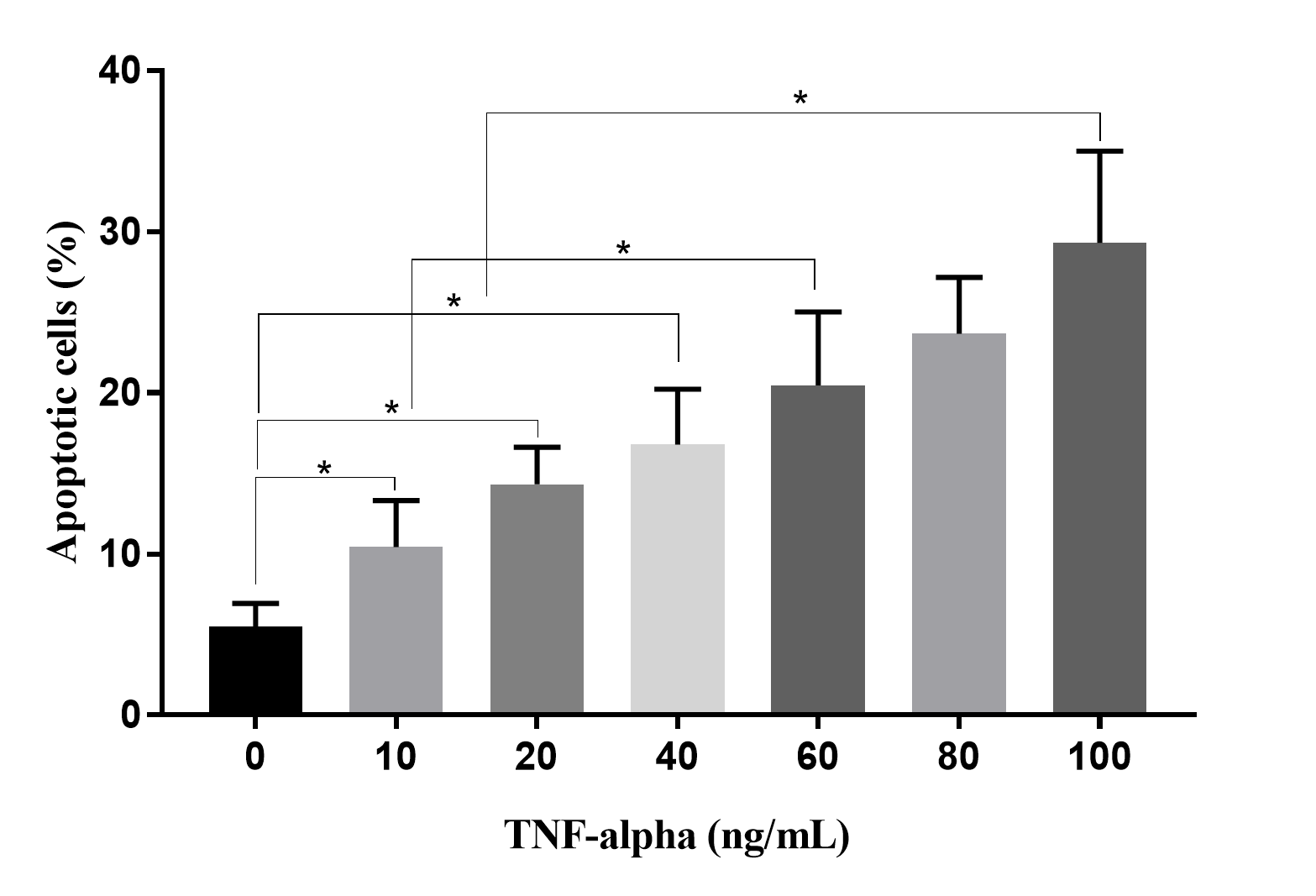
TNF-alpha could cause apoptosis in HUVECs. At a high concentration of TNF-alpha (100 ng/mL), about 30% of HUVECs underwent apoptosis. *p < 0.05.
CM in stress conditions could stimulate angiogenesis but not stressed by TNF-alpha
The results showed that a CM from a normal culture condition (G0; without stress) could support the formation of vascular blood tubes from HUVECs in vitro. Under the microscope, the tubular structure was thin and unclear in this condition (Figure 4A). However, in G1, where ADSCs were treated with TNF-alpha, the HUVEC could not form the vessel structure (Figure 4B). The CM from G2, G3, and G4 could support the blood vessel formation in vitro (Figure 4C, D, and E).
The tubular structures from G0 and G2 were different from standard conditions (positive control) for vessel formation (using M200 medium supplemented with LVES) that could be detected under microscope observation. However, the tubular structures formed in CM from G3 and G4 were similar to that of a positive control (Figure 4).
Dual effects of TNF-alpha on both ADSCs and HUVECs to reduce the angiogenesis potential
The effects of TNF-alpha on VEGF secretion of ADSCs were investigated in different doses (10, 20, 40, 60, 80, and 100 ng/mL). The results revealed that VEGF secretion of ADSCs depends on the concentrations of TNF-alpha (Figure 5). The VEGF concentrations recorded in the CM at treatments of 10 ng/mL and 20 ng/mL of TNF-alpha were higher than that in the CM of control (0 ng/mL of TNF-alpha; 6,600 ± 420 pg/mL and 8,410 ± 502 pg/mL in 10 ng/mL and 20 ng/mL, respectively, of TNF-alpha treatments compared to 5,105 ± 346 pg/mL in control; p < 0.05). However, when TNF-alpha concentrations increased from 40 ng/mL to 100 ng/mL, the VEGF concentrations in the CM gradually reduced. At concentrations of TNF-alpha 80 ng/mL and 100 ng/mL, the VEGF concentrations were lower than that in control (3014 ± 221 pg/mL and 1343 ± 453 pg/mL, respectively, in groups of 80 ng/mL and 100 ng/mL of TNF-alpha treatments compared to 5,105 ± 346 pg/mL in control; p < 0.05).
To evaluate the effects of TNF-alpha in CM on HUVECs, HUVECs were treated with CM collected from culture media supplemented with various concentrations of TNF-alpha. The results revealed that percentages of apoptotic HUVECs gradually increased in response to increased TNF-alpha concentrations (Figure 6). At 0 ng/mL of TNF-alpha, there were 5.50 ± 1.42% apoptotic HUVECs, while this ratio increased to 10.42 ± 2.89 in 10 ng/mL, 14.31 ± 2.31 % in 20 ng/mL, 16.77 ± 3.45 % in 40 ng/mL, 23.67 ± 3.49 % in 80 ng/mL, and 29.32 ± 5.68 % in 100 ng/mL of TNF-alpha (p < 0.05).
Discussion
CM from MSCs is considered a promising cell-free agent for regenerative medicine. As more publications showed that the bio-effects of MSCs are mostly related to their secretomes, CM are of significant interest to researchers and pharmaceutical companies. CM are a combination of various factors such as soluble cytokines, EVs produced by MSCs, and culture media compositions. Under certain stress conditions, MSCs can change the gene expression and production of some cytokines and EVs that cause changes in their bioeffects on target cells. We proposed that when cultured in hypoxia, starvation, or a combination of hypoxia and starvation and TNF-alpha treatment, ADSCs can change the composition of their CM which in turn changes their angiogenic potential. Therefore, in this study, we treated ADSCs in four stress conditions: hypoxia, starvation, a combination of hypoxia and starvation, and TNF-alpha treatment and the assessment of CM on angiogenesis .
In the first experiment, we confirmed that isolated ADSCs express the MSC phenotype as suggested by Dominici (2006)28. Indeed, these cells expressed that the marker profile of MSCs was positive with CD44, CD73, CD90, and CD105 and negative with hematopoietic cells CD14, CD34, CD45, and HLA-DR. Moreover, they could also be induced to mesenchymal cells, including osteoblasts, chondrocytes, and adipocytes .
In the subsequent experiment, the VEGF concentrations in CM collected under various stress conditions were measured. The results showed that ADSCs over-expressed VEGF production only in hypoxia, while in starvation, hypoxia and starvation, and TNF-alpha treatment, ADSC down-regulated VEGF production. Hypoxia can increase VEGF expression at mRNA and protein levels, which was also recorded in some published articles29, 30, 31, 32. Liu. (2013) preconditioned ADSCs in 1% oxygen and demonstrated that in this condition, ADSCs significantly increased the expression of angiogenic factors including VEGF and b-FGF33. This observation was similar to MSCs from the bone marrow34. Lagonda (2018) also showed that at a 3% oxygen level, MSCs from umbilical cord tissue, bone marrow, and adipose tissue significantly increased VEGF synthesis and secretion compared to normoxia35. The mechanism under this observation is related to the activation of HIF-1 alpha caused by hypoxia36. The roles of HIF-1 alpha in angiogenesis are demonstrated in several studies37, 38, 39, 40. Indeed, HIF-1 alpha is a transcription factor regulating the expression of angiogenesis, preventing apoptosis, and inducing migration of cells to ischemia. Hypoxia is known as a condition that induces the expression of HIF-1 alpha38, 39. Besides the effects on VEGF production, hypoxia also causes universal effects on cell proliferation, cell metabolism, cell differentiation, cell apoptosis, and cell migration. In some studies, MSCs from the umbilical cord41, adipose tissue32, and urine stem cells42 could increase cell proliferation in hypoxic conditions (1.5% — 5% oxygen)41, while in other studies, MSCs could reduce their proliferation in hypoxic condition43.
Starvation is also a common kind of stress that can change MSCs’ characteristics. In this study, we found that starvation reduced VEGF production. This observation was also reported by Chua . (2013)23 and Rezaie . (2018)44. The reason for this property has not been explained in the previous publications. This study also has not figured out why VEGF production reduced in starvation. We proposed that this characteristic could relate to cell proliferation that significantly reduces the stress of starvation conditions. The considerable decrease in VEGF production in starvation could cause a significant reduction of VEGF when ADSCs are treated with both the stresses of hypoxia and starvation.
The effects of TNF-alpha on the angiogenic potential of MSCs are also controversial. A few studies suggested that TNF-alpha could stimulate VEGF production in MSCs45, 46, 47, while others demonstrated that TNF-alpha is an inhibitor of VEGF production in MSCs48. In this study, the effects of TNF-alpha depended on its dose. At a low dose (below 40 ng/mL), TNF-alpha could stimulate ADSCs to produce VEGF; however, at a higher dose, TNF-alpha inhibited VEGF production.
However, the reduction of VEGF in the CM did not directly affect the failure of CM from TNF-alpha-stressed ADSC culture in the blood vessel formation of HUVECs. Indeed, the results indicate that in hypoxia (G2) and hypoxia combined with starvation (G4), the levels of VEGF were similar to that of TNF-alpha treatment (G1), but their CM also supported blood vessel formation of HUVECs . Based on this finding, we propose that the remaining TNF-alpha in CM had a direct negative effect on the HUVECs and inhibited blood vessel formation. Under a microscope, we found that almost all HUVECs could enter an apoptotic phase. Therefore we propose that TNF-alpha could cause apoptosis in HUVECs in the next experiment.
To demonstrate that CM from TNF-alpha-stressed ADSC culture depends on reducing VEGF and that the percentage of apoptotic HUVECs directly depends on the concentration of TNF-alpha, CM derived from 100 ng/mL of TNF-alpha culture medium caused to 29.32 ± 5.68 % apoptotic HUVECs after 48 h of treatment. Hence, the effects of TNF-alpha in culture medium affect both ADSCs and HUVECs. Furthermore, although it can enhance VEGF production at low doses, TNF-alpha directly drives HUVECs to apoptosis; the blood vessel formation assay confirmed this analysis.
Blood vessels could be formed from HUVECs in CM from control (without stress), hypoxia, starvation, and starvation and hypoxia but not in the TNF-alpha stress group. The VEGF concentration can affect the angiogenic potential of these CM but does not depend on their concentration. In the starvation stress or starvation combined with hypoxia conditions, the VEGF concentration was also low compared to that in TNF-alpha stress. However, the blood vessel matrix could be formed . This implies that extra TNF-alpha in the CM of TNF-alpha stress ADSC culture inhibited the blood vessel formation of HUVECs. Moreover, TNF-alpha also changed the EV synthesis and production. Ahn . (2018) suggested that MSCs can produce EVs to carry and transfer VEGF to injured cells in models of neonatal hyperoxic lung injuries49.
Conclusions
CM from preconditioned MSC cultures are considered new components of regenerative medicine. CM can be used as free-cell therapy; they open up new opportunities for tissue healing. In this study, we found that CM from various culture conditions can directly affect VEGF production of ADSCs which in turn can change the angiogenic potential of CM. In hypoxic culture conditions, ADSCs strongly produce and secrete VEGF in the CM, while in starvation culture conditions as well as TNF-alpha treatment, ADSCs significantly reduced VEGF production. However, it seems that not only did VEGF in CM affect blood vessel formation of HUVECs but also had other effects. The extra TNF-alpha in CM of TNF-alpha treatment negatively affects the blood vessel formation by promoting apoptosis of HUVECs. Other mechanisms underlying this observation that can relate to the EVs and exosome synthesis and production of ADSCs treated with TNF-alpha have not been evaluated in this study.
Abbreviations
ADSC: Adipose derived stem cell
CM: Conditioned medium
HGF: Hepatic growth factor
HUVEC: Human umbilical vein endothelial cell
IGF: Insulin-like growth factor
IL: Interleukin
MCP: Monocyte chemotactic factor
MSC: Mesenchymal stem cell
SDF: Stromal cell-derived factor
TNF: Tissue necrosis factor
VCAM: Vascular cell adhesion
VEGF: Vascular endothelial growth factor
Acknowledgments
Authors thank Van Hanh General Hospital, Ho Chi Minh City, Viet Nam approved to use the adipose tissues from donors for the study.
Author’s contributions
Phuc Van Pham contributed to the conceptualization and design of the study, the acquisition, analysis and interpretation of data. Ngoc Bich Vu, Binh Thanh Vu and Khanh Nha Nguyen were for drafting the article and revising the article critically for important intellectual content. All authors read and approved the final manuscript.
Funding
This work is supported by Vietnam National University Ho Chi Minh City, No.TX2019-18-02.
Availability of data and materials
Data and materials used and/or analyzed during the current study are available from the corresponding author on reasonable request.
Ethics approval and consent to participate
Not applicable.
Consent for publication
Not applicable.
Competing interests
The authors declare that they have no competing interests.