Hypoxia promotes adipose-derived stem cell proliferation via VEGF
Abstract
Adipose-derived stem cells (ADSCs) are a promising mesenchymal stem cell source with therapeutic applications. Recent studies have shown that ADSCs could be expanded in vitro without phenotype changes. This study aimed to evaluate the effect of hypoxia on ADSC proliferation in vitro and to determine the role of vascular endothelial growth factor (VEGF) in ADSC proliferation. ADSCs were selectively cultured from the stromal vascular fraction obtained from adipose tissue in DMEM/F12 medium supplemented with 10% fetal bovine serum and 1% antibiotic-antimycotic. ADSCs were cultured under two conditions: hypoxia (5% O2) and normal oxygen (21% O2). The effects of the oxygen concentration on cell proliferation were examined by cell cycle and doubling time. The expression of VEGF was evaluated by the ELISA assay. The role of VEGF in ADSC proliferation was studied by neutralizing VEGF with anti-VEGF monoclonal antibodies. We found that the ADSC proliferation rate was significantly higher under hypoxia compared with normoxia. In hypoxia, ADSCs also triggered VEGF expression. However, neutralizing VEGF with anti-VEGF monoclonal antibodies significantly reduced the proliferation rate. These results suggest that hypoxia stimulated ADSC proliferation in association with VEGF production.
Introduction
Adipose-derived stem cells (ADSCs) have been widely used in many clinical fields. ADSCs exhibit the mesenchymal stem cell (MSC) phenotype Bourin et al., 2013Zimmerlin et al., 2013, as evidenced by the expression of MSC markers such as CD44, CD73, CD90 and CD105. They can successfully differentiate into adipocytes, osteoblasts and chondrocytes, and have been trans-differentiated into other mesoderm cell types such as insulin producing cells Karaoz et al., 2013Moshtagh et al., 2013, hepatocytes Aurich et al., 2009Okura et al., 2010 and neuronal-like cells Cardozo et al., 2010Rezanejad et al., 2014. ADSC transplantation ameliorated some diseases such as cranio-maxillofacial hard-tissue defects Sandor et al.,2014, non-revascularizable critical limb ischemia Bura et al., 2014, acute myocardial infarction and heart failure Panfilov et al., 2013, complex perianal fistula in Crohn’s disease de la Portilla et al., 2013Garcia-Olmo et al., 2009, and chronic myocardial ischemia Qayyum et al., 2012.
In recent years, hypoxia has been applied to cultured ADSCs in vitro. In fact, hypoxia was demonstrated as a stimulator of ADSC proliferation, but its effects did not change the ADSC phenotype Fotia et al., 2015Wan Safwani et al., 2016. Hypoxia has also been demonstrated as a protector from senescence Lee et al.,2015, genomic stability inducer Bigot et al., 2015, maintainer of stemness Fotia et al., 2015Petrangeli et al., 2016Wan Safwani et al., 2016, and enhancer of viability, motility and tropism Feng et al., 2015. These hypoxia effects have been demonstrated through stimulation of hypoxia-inducible factor 1-α (HIF-1α) expression Kakudo et al., 2015, generation of reactive oxygen species (ROS) and downstream phosphorylation of platelet-derived growth factor receptor β (PDGFRβ), extracellular signal-regulated kinases 1/2 (ERK1/2) and Akt Kang et al., 2014Kim et al., 2011.
This study aimed to identify another mechanism of hypoxia that stimulates ADSC proliferation. Our findings provide new insight into controlling ADSCs in research and medical applications.
Materials and Methods
Isolation of stromal vascular fractions (SVFs)
Adipose tissues were collected from the abdomen by aspiration with a suitable needle from donors who signed a consent form. Approximately 50–100 mL of lipoaspirate were collected in two 50 mL sterile syringes. The syringes were stored in a sterile box at 2–8°C and were immediately transferred to the laboratory.
The SVF was isolated from adipose tissues using a Cell Extraction Kit (BioMedFactory, Ho Chi Minh, Vietnam) according to the manufacturer’s instructions. Briefly, adipose tissue was mixed with an enzyme solution containing collagenase at 37°C for 15 min. Then, the cell suspension obtained was centrifuged at 3000 ×g for 10 min, and the SVF was obtained as the pellet. The pellet was washed with PBS to remove any residual enzymes, and resuspended in PBS to determine cell quantity and viability using an automatic cell counter (NucleoCounter, Chemometec, Denmark).
SVF culture
SVF samples were cultured in MSCCult medium (BioMedFactory) containing DMEM/F12 supplemented with antibiotic-antimycotic and 10% fetal bovine serum (FBS). The cells were plated at 5 × 104 cells/mL in T-75 flasks (Corning) and incubated at 37°C with 5% CO2. After 3 days of incubation, 6 mL of fresh medium was added to each flask. After 7 days, the medium was replaced with 12 mL of fresh media. The medium was subsequently replaced every 3 days until the cells reached 70–80% confluence, and then they were subcultured.
ADSC phenotyping and characterization
Cell markers were analyzed following a previously published protocol. Briefly, cells were washed twice with PBS containing 1% bovine serum albumin (BSA). The cells were then stained with anti-CD14-FITC, anti-CD44-PE, anti-CD45-FITC, anti-CD105-FITC, anti-CD90-PE and anti-HLA-DR-FITC antibodies (all purchased from BD Biosciences, San Jose, CA, USA). Stained cells were analyzed by a FACSCalibur flow cytometer (BD Biosciences). Isotype controls were used in all analyses.
For differentiation into adipogenic cells, ADSCs were treated as described previously. Briefly, cells were plated at 1 × 104 cells/well in 24-well plates. At 70% confluence, the cells were cultured for 21 days in DMEM/F12 containing 0.5 mM 3-isobutyl-1-methylxanthine, 1 nM dexamethasone, 0.1 mM indomethacin and 10% FBS (all purchased from Sigma-Aldrich, St Louis, MO, USA). Adipogenic differentiation was evaluated by observing lipid droplets in cells under a microscope. For differentiation into osteogenic cells, ADSCs were plated at 1 × 104 cells/well in 24-well plates. At 70% confluence, the cells were cultured for 21 days in DMEM/F12 containing 10% FBS, 1 × 10-7 M dexamethasone, 50 μM ascorbic acid-2 phosphate and 10 mM β-glycerol phosphate (all purchased from Sigma-Aldrich). Osteogenic differentiation was confirmed by Alizarin red staining.
Normoxia and hypoxia culture
To determine the effect of hypoxia on cell proliferation, the ADSC were seeded at a density of 1000 cells/wellin 96-well plates (Costar, Acton, MA, USA) and culturedat 5% or 20% oxygen. On days 2, 4, 6, 8, 10, 12, 14 and 16, the cells were lysed in 200 μL 0.02% sodium dodecyl sulphate (SDS) in DNase-free water, after which the cell number in thesamples was determined using a Pico-GreendsDNAquantitation kit (Invitrogen). The number of cells was calculatedusing a theoretical value of 6.6 pg DNA/cell. The experiment was performed three times, each in quadruplicate.
Similarly, this procedure was repeated in a tri-gas incubator in which the oxygen concentration was controlled to 5%.
Cell cycle
Cell cycle analysis was carried out according to the following protocols. Cells from each group were washed twice with PBS and fixed in cold 70% ethanol for at least 3 h at 4°C. Cells were then washed twice with PBS and stained with 1 mL of propidium iodide (PI; 20 μg/mL). RNase A (10 μg/mL) was added to the samples and incubated for 3 h at 4°C. Stained cells were analyzed by flow cytometry using CellQuest Pro software (BD Biosciences, Franklin Lakes, NJ, USA).
Effects of neutralizing VEGF
ADSCs were cultured in E-plates and the eXCELLI-gence system was used to evaluate cell proliferation. The culture medium was replaced with culture medium supplemented with mAbs against VEGF (clone 4.6.1), VEGFR-1 (clone 6.12) or VEGFR-2 (clone IMC1C11). These mAbs were freshly added to the medium at 1 μg per 1 mL of medium. The cell proliferation, doubling time and cell cycle were evaluated similar to in the normal condition (without mAbs).
Expression of VEGFR-1, VEGFR-2 and PDGFRβ
The expression of VEGFR-1, VEGFR-2 and PDGFRβ was analyzed following protocol. Briefly, cells were washed twice with PBS containing 1% BSA. The cells were then stained with anti-VEGFR-1-FITC, anti-VEGFR-2-FITC or anti-PDGFRβ-PE (all purchased from Abcam). Stained cells were analyzed by a FACS-Calibur flow cytometer (BD Biosciences). Isotype controls were used in all analyses.
Statistical analysis
Significant differences between mean values were assessed by t-tests and analysis of variance (ANOVA). P< 0.05 was considered statistically significant. All data were analyzed by Prism 6 software.
Results
ADSC isolation and culture
Similar to previous studies, SVFs from adipose tissue easily attached to the flask surface. After 24 h of incubation at 37°C, some cells attached and exhibited spindle shapes ( Figure 1 ). These cells continuously proliferated and appeared as fibroblast-like cells after 72 h of incubation. After 7 days, the primary culture reached about 70% confluence. The cells were subcultured to a third passage and used in further experiments.
ADSC characterization and in vitro differentiation
To examine whether these ADSC cells show MSC phenotypes, the MSC marker profile of these cells was assayed. The ADSC candidates strongly expressed markers such as CD44, CD90 and CD105 ( Figure 2 ), while they did not express CD14, CD45 and HLA-DR. Moreover, they successfully differentiated into three kinds of mesoderm cells including adipocytes, which showed positive Red Oil staining, osteoblasts, which showed positive Alizarin Red staining, and chondroblasts, which showed positive Safranin O ( Figure 2 ).
ADSC proliferation is different in hypoxia and normoxia
Based on the proliferation curve, we found that after 6 days, the proliferation of ADSCs was different between hypoxia and normoxia. The doubling time significantly decreased in hypoxia-cultured ADSCs compared with normoxia-cultured ADSCs at the 6th day and 10th day. At the 1st day the doubling time of ADSCs in the two groups was not different (43.5 h vs. 40.11 h in hypoxia and normoxia, respectively), however, after 6 days of culture the doubling time under hypoxia decreased to 40.11 h at 1st day to 30.0 h at 6th day, respectively. These values were significantly different from those under normoxia (43.5 h at 1st day and 40.5 at the 6thday). These results were confirmed by a cell cycle assay. After 1st day in culture, the ADSC’s cell cycle hardly changed under the two conditions. However, the differences became clear after 6th day. The proportion of cells in S phase significantly decreased in hypoxia-cultured ADSCs at 6th day compared to 1st day (29.33 ± 3.06% at 6th day compared to 39.01 ± 2.52% at the 1st day), while the change was not significant in normoxia-cultured ADSCs (40.50 ± 0.29% in 6th day compared to 38.88 ± 1.21% in the 1st day). Figure 3
ADSCs strongly express VEGF under hypoxia compared with normoxia
The concentration of VEGF in conditioned medium under hypoxia was significantly higher than that under normoxia. The results showed that the VEGF concentration in conditioned medium under hypoxia increased at 6th day compared with 1st day ( Figure 4 )(from 671 ± 43.14 pg/mL at 1st day to 1223 ± 50.44 pg/mL at 6thday).While this concentration was non-significantly increased in the normoxia group (from 624 ± 42.44 pg/mL at 1st day to at 724.3 ± 33.94 pg/mL 6th day).
The expression of VEGFR-1, VEGFR-2 and PDGFRβ
Using flow cytometry, VEGFR-1 and VEGFR-2 expression was evaluated at 48 hand 72 h in the hypoxiatreated culture. The results showed that some ADSCs expressed VEGFR-1 and VEGFR-2 at a low level (less than 1%), while more than 95% of ADSCs expressed PDGFRβ ( Figure 5 ).
ADSC proliferation decreases in culture medium supplemented with anti-VEGF or anti-VEGFR mAbs
ADSC proliferation under hypoxia decreased in culture medium supplemented with anti-VEGF or anti-VEGFR mAbs compared with under hypoxia without mAbs. Both doubling time and cell cycle supported this observation. The proliferation rate of ADSCs in culture medium supplemented with anti-VEGF or anti-VEGFR mAbs was significantly decreased compared with that in normal culture medium (without anti-VEGF or anti-VEGFR mAbs) under hypoxia. However, ADSCs in culture medium supplemented with anti-VEGF or anti-VEGFR mAbs still proliferated more rapidly than under normoxia.
As shown in Figure 6 , anti-VEGF or anti-VEGFR mAbs-supplemented medium decreased the growth of ADSCs compared with normal medium (without anti-VEGF or anti-VEGFR mAbs). The doubling time of these ADSCs was non-significantly between hypoxia and normoxia at 6th day (35.67 ± 0.67 hrs in hypoxia and 40.50 ± 0.29 hrs in normoxia). The proportion of cells in S phase under these conditions also was nonsignificantly at 6thday (37.33 ± 2.51% in hypoxia, and 41.33 ± 2.08% in normoxia, espectively).
Discussion
ADSCs are a promising candidate for clinical applications. They are easily derived from adipose tissue, with strong proliferation potential. Compared with other sources of MSCs such as bone marrow, umbilical cord and umbilical cord blood, ADSCs have a shorter doubling time. Therefore, numerous studies on ADSCs, especially on ADSC in vitro proliferation, have been performed to date. To date, hypoxia has been applied on stem cell cultures including ADSC culture. This study aimed to evaluate the effects of hypoxia on ADSC proliferation.
First, ADSCs were successfully isolated and confirmed by usual methods and assays. Similarly to previous studies, the obtained ADSCs fully exhibited several MSCs characteristics, including adherence to the surface of plastic flasks with a fibroblast-like shape; they also successfully differentiated into three kinds of mesoderm cells including adipocytes, osteoblasts and chondroblasts. Flow cytometry analysis with surface markers revealed that they expressed particular markers of MSCs, such as CD44, CD90 and CD105, while hematopoietic markers such as CD14, CD45 and HLADR were not detected.
These MSCs were used in the following experiments. These cells demonstrated higher proliferation under hypoxia compared with normoxia. Further assays of doubling time and cell cycle supported this finding. In fact, the doubling time significantly decreased under hypoxia compared with normoxia. Cell cycle analysis also showed that hypoxia significantly decreased the percentage of ADSCs in phase S. These findings showed that hypoxia stimulated the cells to transition into the phase S, reduced the proportion of cells in S phase, and therefore stimulated cell proliferation.
To determine why ADSCs proliferated rapidly under hypoxia compared with normoxia, the supernatants of the cell cultures under hypoxia and normoxia were collected and the concentration of VEGF was analyzed. The results showed that there was a significant increase in VEGF expression in the supernatant of hypoxia-cultured cells compared with normoxiacultured cells, suggesting that the strong proliferation of ADSCs is relate to the VEGF concentration. Thus, we think that hypoxia stimulates ADSCs to produce and secret VEGF into the supernatant, and that VEGF acts as an autocrine factor that stimulates ADSC proliferation.
Previous studies have also shown that under hypoxia, ADSCs produced more VEGF He et al., 2015Liu et al., 2013Rinkinen et al., 2015. In fact, with a high concentration of VEGF, the ADSC-conditioned medium exhibited positive effects on some degenerative diseases such as myocardial infarction in rats He et al.,2015 and diabetic erectile dysfunction Wang et al., 2015. Particularly, these studies have shown that the main effect of hypoxia-pretreated ADSCs is augmentation of angiogenesis He et al., 2015Hsiao et al.,2013Liu et al., 2013Wang et al., 2015.
However, we hypothesized that the effects of ADSC proliferation are associated with autocrine effects of VEGF on ADSCs. Therefore, we evaluated the expression of VEGF receptors in hypoxia-treated ADSCs. However, both flow cytometry and real-time RT PCR of VEGFR-1 and VEGFR-2 showed that these cells hardly expressed these receptors. But, in another assay the proliferation of the hypoxia-treated ADSCs decreased after adding anti-VEGF mAbs to neutralize the VEGF effects, although, the hypoxia-treated ADSCs with anti-VEGF mAb still proliferated more rapidly than ADSCs under normoxia.
Taken together, we hypothesized that there are at least two pathways involved in ADSC proliferation under hypoxia: the first one is VEGF-dependent, and the second is VEGF-independent. In fact, the effects of hypoxia on ADSC proliferation have been demonstrated to involve activation of the HIF-1α pathway Kakudo et al., 2015. Using ERK and Akt inhibitors, Kakudo et al., 2015 have determined that the effects of hypoxia on ADSC proliferation could be blocked, and that HIF-1α knockdown by siRNA partially inhibited the hypoxia-induced FGF-2 expression in ADSCs Kakudo et al., 2015. In the second pathway, we speculated that VEGF affects ADSCs via receptors other than VEGFR-1 and VEGR-2. Thus, we evaluated PDGFRβ expression in hypoxic ADSCs and showed that ADSCs highly expressed this receptor. In previous reports, PDGFRβ has been demonstrated as a facultative receptor for VEGF Ball et al., 2007Mabry et al., 2010Pfister et al.,2012. Ball et al., 2007 have shown that VEGF-A could bind to both PDGFRα and PDGFRβ, inducing tyrosine phosphorylation. When inhibited, VEGF-A-induced MSC migration and proliferation were suppressed Ball et al., 2007.
In combination with previous studies, we propose that hypoxia can stimulate ADSC proliferation via two mechanisms: hypoxia-stimulated expression of HIF-1α activates this signaling pathway, which induces VEGF secretion into the conditioned medium. The autocrine VEGF effect on ADSCs significantly triggers ADSC proliferation by efficiently reducing the proportion of S phase cells and reducing the doubling time.
Conclusion
ADSCs are promising stem cells for clinical applications. Hypoxia treatment was used to trigger ADSC proliferation. This study showed that hypoxia strongly stimulated ADSC proliferation. Under hypoxia, ADSCs were stimulated to produce VEGF, however, VEGF may have an autocrine effect on ADSCs. Secreted VEGF stimulated ADSC proliferation via PDGFRβ. These findings will contribute to understanding stem cell proliferation under hypoxia as well as application of this mechanism to control stem cell proliferation and differentiation.
References
-
H.
Aurich,
M.
Sgodda,
P.
Kaltwasser,
M.
Vetter,
A.
Weise,
T.
Liehr,
M.
Brulport,
J.G.
Hengstler,
M.M.
Dollinger,
W.E.
Fleig.
Hepatocyte differentiation of mesenchymal stem cells from human adipose tissue in vitro promotes hepatic integration in vivo. Gut.
2009;
58
:
570-581
.
-
S.G.
Ball,
C.A.
Shuttleworth,
C.M.
Kielty.
Vascular endothelial growth factor can signal through platelet-derived growth factor receptors. J Cell Biol.
2007;
177
:
489-500
.
-
N.
Bigot,
A.
Mouche,
M.
Preti,
S.
Loisel,
M.L.
Renoud,
R.
Le Guevel,
L.
Sensebe,
K.
Tarte,
R.
Pedeux.
Hypoxia Differentially Modulates the Genomic Stability of Clinical-Grade ADSCs and BM-MSCs in Long-Term Culture. Stem Cells.
2015;
33
:
3608-3620
.
-
P.
Bourin,
B.A.
Bunnell,
L.
Casteilla,
M.
Dominici,
A.J.
Katz,
K.L.
March,
H.
Redl,
J.P.
Rubin,
K.
Yoshimura,
J.M.
Gimble.
Stromal cells from the adipose tissue-derived stromal vascular fraction and culture expanded adipose tissue-derived stromal/stem cells: a joint statement of the International Federation for Adipose Therapeutics and Science (IFATS) and the International Society for Cellular Therapy (ISCT). Cytotherapy.
2013;
15
:
641-648
.
-
A.
Bura,
V.
Planat-Benard,
P.
Bourin,
J.S.
Silvestre,
F.
Gross,
J.L.
Grolleau,
B.
Saint-Lebese,
J.A.
Peyrafitte,
S.
Fleury,
M.
Gadelorge.
Phase I trial: the use of autologous cultured adiposederived stroma/stem cells to treat patients with non-revascularizable critical limb ischemia. Cytotherapy.
2014;
16
:
245-257
.
-
A.
Cardozo,
M.
Ielpi,
D.
Gomez,
P.
Argibay.
Differential expression of Shh and BMP signaling in the potential conversion of human adipose tissue stem cells into neuron-like cells in vitro. Gene Expr.
2010;
14
:
307-319
.
-
F.
Portilla,
F.
Alba,
D.
Garcia-Olmo,
J.M.
Herrerias,
F.X.
Gonzalez,
A.
Galindo.
Expanded allogeneic adipose-derived stem cells (eASCs) for the treatment of complex perianal fistula in Crohn’s disease: results from a multicenter phase I/IIa clinical trial. Int J Colorectal Dis.
2013;
28
:
313-323
.
-
Y.
Feng,
M.
Zhu,
S.
Dangelmajer,
Y.M.
Lee,
O.
Wijesekera,
C.X.
Castellanos,
A.
Denduluri,
K.L.
Chaichana,
Q.
Li,
H.
Zhang.
Hypoxia-cultured human adipose-derived mesenchymal stem cells are non-oncogenic and have enhanced viability, motility, and tropism to brain cancer. Cell Death Dis.
2015;
6
:
e1797
.
-
C.
Fotia,
A.
Massa,
F.
Boriani,
N.
Baldini,
D.
Granchi.
Hypoxia enhances proliferation and stemness of human adipose-derived mesenchymal stem cells. Cytotechnology.
2015;
67
:
1073-1084
.
-
D.
Garcia-Olmo,
D.
Herreros,
I.
Pascual,
J.A.
Pascual,
E.
Del-Valle,
J.
Zorrilla,
P.
De-La-Quintana,
M.
Garcia-Arranz,
M.
Pascual.
Expanded adipose-derived stem cells for the treatment of complex perianal fistula: a phase II clinical trial. Dis Colon Rectum.
2009;
52
:
79-86
.
-
J.
He,
Y.
Cai,
L.M.
Luo,
H.B.
Liu.
Hypoxic adipose mesenchymal stem cells derived conditioned medium protects myocardial infarct in rat. Eur Rev Med Pharmacol Sci.
2015;
19
:
4397-4406
.
-
S.T.
Hsiao,
Z.
Lokmic,
H.
Peshavariya,
K.M.
Abberton,
G.J.
Dusting,
S.Y.
Lim,
R.J.
Dilley.
Hypoxic conditioning enhances the angiogenic paracrine activity of human adipose-derived stem cells. Stem Cells Dev.
2013;
22
:
1614-1623
.
-
N.
Kakudo,
N.
Morimoto,
T.
Ogawa,
S.
Taketani,
K.
Kusumoto.
Hypoxia Enhances Proliferation of Human Adipose-Derived Stem Cells via HIF-1a Activation. PLoS One.
2015;
10
:
e0139890
.
-
S.
Kang,
S.M.
Kim,
J.H.
Sung.
Cellular and molecular stimulation of adipose-derived stem cells under hypoxia. Cell Biol Int.
2014;
38
:
553-562
.
-
E.
Karaoz,
A.
Okcu,
Z.S.
Unal,
C.
Subasi,
O.
Saglam,
G.
Duruksu.
Adipose tissue-derived mesenchymal stromal cells efficiently differentiate into insulin-producing cells in pancreatic islet microenvironment both in vitro and in vivo. Cytotherapy.
2013;
15
:
557-570
.
-
J.H.
Kim,
S.H.
Park,
S.G.
Park,
J.S.
Choi,
Y.
Xia,
J.H.
Sung.
The pivotal role of reactive oxygen species generation in the hypoxia-induced stimulation of adipose-derived stem cells. Stem Cells Dev.
2011;
20
:
1753-1761
.
-
J.
Lee,
J.S.
Byeon,
K.S.
Lee,
N.Y.
Gu,
G.B.
Lee,
H.R.
Kim,
I.S.
Cho,
S.H.
Cha.
Chondrogenic potential and anti-senescence effect of hypoxia on canine adipose mesenchymal stem cells. Vet Res Commun.
2015
.
-
L.
Liu,
J.
Gao,
Y.
Yuan,
Q.
Chang,
Y.
Liao,
F.
Lu.
Hypoxia preconditioned human adipose derived mesenchymal stem cells enhance angiogenic potential via secretion of increased VEGF and bFGF. Cell Biol.
2013;
Int37
:
551-560
.
-
R.
Mabry,
D.G.
Gilbertson,
A.
Frank,
T.
Vu,
D.
Ardourel,
C.
Ostrander,
B.
Stevens,
S.
Julien,
S.
Franke,
B.
Meengs.
A dual-targeting PDGFRbeta/VEGF-A molecule assembled from stable antibody fragments demonstrates anti-angiogenic activity in vitro and in vivo. MAbs.
2010;
2
:
20-34
.
-
P.R.
Moshtagh,
S.H.
Emami,
A.M.
Sharifi.
Differentiation of human adipose-derived mesenchymal stem cell into insulin-producing cells: an in vitro study. J Physiol Biochem.
2013;
69
:
451-458
.
-
H.
Okura,
H.
Komoda,
A.
Saga,
A.
Kakuta-Yamamoto,
Y.
Hamada,
Y.
Fumimoto,
C.M.
Lee,
A.
Ichinose,
Y.
Sawa,
A.
Matsuyama.
Properties of hepatocyte-like cell clusters from human adipose tissue-derived mesenchymal stem cells. Tissue Eng Part C Methods.
2010;
16
:
761-770
.
-
I.A.
Panfilov,
R.
de Jong,
S.
Takashima,
H.J.
Duckers.
Clinical study using adipose-derived mesenchymal-like stem cells in acute myocardial infarction and heart failure. Methods Mol Biol.
2013;
1036
:
207-212
.
-
E.
Petrangeli,
G.
Coroniti,
A.T.
Brini,
L.
de Girolamo,
D.
Stanco,
S.
Niada,
G.
Silecchia,
E.
Morgante,
C.
Lubrano,
M.A.
Russo.
Hypoxia Promotes the Inflammatory Response and Stemness Features in Visceral Fat Stem Cells From Obese Subjects. J Cell Physiol.
2016;
231
:
668-679
.
-
C.
Pfister,
H.
Pfrommer,
M.S.
Tatagiba,
F.
Roser.
Vascular endothelial growth factor signals through platelet-derived growth factor receptor beta in meningiomas in vitro. Br J Cancer.
2012;
107
:
1702-1713
.
-
A.A.
Qayyum,
M.
Haack-Sorensen,
A.B.
Mathiasen,
E.
Jorgensen,
A.
Ekblond,
J.
Kastrup.
Adipose-derived mesenchymal stromal cells for chronic myocardial ischemia (MyStromalCell Trial): study design. Regen Med.
2012;
7
:
421-428
.
-
H.
Rezanejad,
Z.S.
Soheili,
F.
Haddad,
M.M.
Matin,
S.
Samiei,
A.
Manafi,
H.
Ahmadieh.
In vitro differentiation of adiposetissue-derived mesenchymal stem cells into neural retinal cells through expression of human PAX6 (5a) gene. Cell Tissue Res.
2014;
356
:
65-75
.
-
J.
Rinkinen,
J.
Lisiecki,
E.
Oluwatobi,
J.
Peterson,
S.
De La Rosa,
K.
Ranganathan,
S.C.
Wang,
P.S.
Cederna,
B.
Levi.
Role of anatomical region and hypoxia on angiogenic markers in adiposederived stromal cells. J Reconstr Microsurg.
2015;
31
:
132-138
.
-
G.K.
Sandor,
J.
Numminen,
J.
Wolff,
T.
Thesleff,
A.
Miettinen,
V.J.
Tuovinen,
B.
Mannerstrom,
M.
Patrikoski,
R.
Seppanen,
S.
Miettinen.
Adipose stem cells used to reconstruct 13 cases with cranio-maxillofacial hard-tissue defects. Stem Cells Transl Med.
2014;
3
:
530-540
.
-
W.K.
Wan Safwani,
C.W.
Wong,
K.W.
Yong,
J.R.
Choi,
N.A.
Mat Adenan,
S.Z.
Omar,
W.A.
Wan Abas,
B.
Pingguan-Murphy.
The effects of hypoxia and serum-free conditions on the stemness properties of human adipose-derived stem cells. Cytotechnology.
2016
.
-
X.
Wang,
C.
Liu,
S.
Li,
Y.
Xu,
P.
Chen,
Y.
Liu,
Q.
Ding,
W.
Wahafu,
B.
Hong,
M.
Yang.
Hypoxia precondition promotes adipose-derived mesenchymal stem cells based repair of diabetic erectile dysfunction via augmenting angiogenesis and neuroprotection. PLoS One.
2015;
10
:
e0118951
.
-
L.
Zimmerlin,
V.S.
Donnenberg,
J.P.
Rubin,
A.D.
Donnenberg.
Mesenchymal markers on human adipose stem/progenitor cells. Cytometry A.
2013;
83
:
134-140
.
Comments
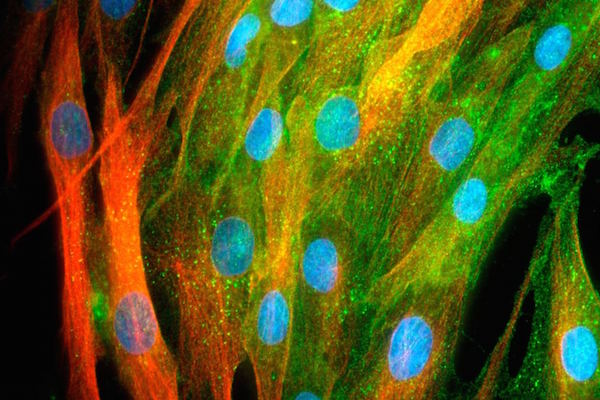
Downloads
Article Details
Volume & Issue : Vol 3 No 01 (2016)
Page No.: 476-482
Published on: 2016-02-29
Citations
Copyrights & License

This work is licensed under a Creative Commons Attribution 4.0 International License.
Search Panel
Pubmed
Google Scholar
Pubmed
Google Scholar
Pubmed
Search for this article in:
Google Scholar
Researchgate
- HTML viewed - 7149 times
- Download PDF downloaded - 1608 times
- View Article downloaded - 6 times