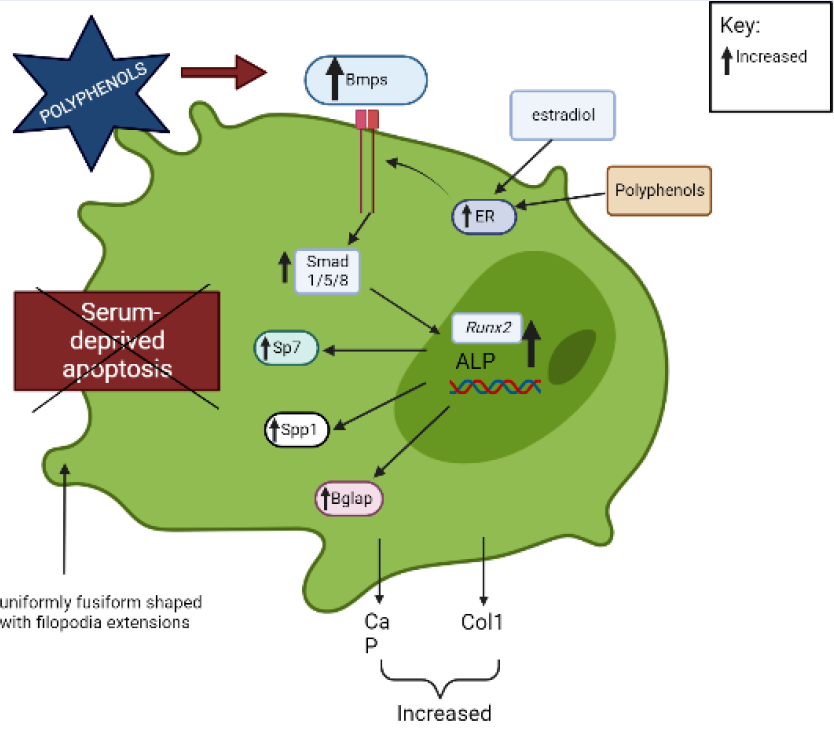
Potential Effects of Polyphenols on Osteoblast and Osteoclast Culture
- Biomedicine Programme, School of Health Sciences, Universiti Sains Malaysia, Health Campus, 16150 Kubang Kerian, Kelantan, Malaysia
- School of Dental Sciences, Universiti Sains Malaysia, Health Campus, 16150 Kubang Kerian, Kelantan, Malaysia
- Department of Pharmacology, Faculty of Medicine, Universiti Kebangsaan Malaysia, Jalan Yaacob Latif, Bandar Tun Razak, 56000 Cheras, Kuala Lumpur, Malaysia
Abstract
Bone tissue undergoes constant remodeling by reducing the accumulation of bone damage and retaining the mechanical strength of bones to sustain both structural integrity and bone density. There are two main specialized cells involved in the bone-remodeling process, osteoblasts (OBs) and osteoclasts (OCs), which are responsible for new bone formation and aged bone resorption, respectively. The proper balancing act between bone resorption by OCs and bone deposition by OBs is essential for the active and dynamic process of bone remodeling. Polyphenols are a group of phytochemicals that are found in plants. Due to their bioactive components, like flavonoids, phenolic acids, and stilbenes, medicinal plants have long been pursued in the drug development process. Many medicinal plant extracts have been found to improve bone health. To provide more applicable preclinical research results, scientists have concentrated on developing in vitro models of bone cells by utilizing cell lines or primary cells. However, OBs and OCs do not act independently of one another, and various communication pathways between them have been discovered. This review summarizes the relevant data from existing studies on the effects of polyphenols on OBs and OCs using monocultures; these studies can be further enriched using co-culture, which represents an experimental system closer to the in vivo conditions than monoculture, allowing realistic cell– cell interactions. This information will be valuable for the development of new pharmaceutical and nutraceutical agents to treat and manage bone diseases.
Introduction
Bone remodeling is the dynamic process of bone formation and resorption in vertebrates to maintain bone volume and calcium homeostasis1, 2. Osteoclasts (OCs) and osteoblast (OBs) are the two main specialized cells involved in the bone remodeling process through which the old or damaged bones are resorbed by OCs (bone resorption) and new bone is formed by OBs (bone formation)3, 4. The proper balancing act between bone resorption by OCs and bone deposition by OBs is important in the maintenance of healthy bones2, 4.
OBs and OCs work in balance, and a dysregulated interplay or imbalance between these cell types may result in implications on the bone that vary from fractures that do not heal effectively to major conditions, such as osteoporosis or in rare cases, osteopetrosis5, 6, 7, 8. The National Institutes of Health Consensus Development Panel defines osteoporosis as “a skeletal disorder characterized by compromised bone strength predisposing a person to an increased risk of fracture”9, 10. Osteoporosis is a silent disease that is often undiagnosed11, 12. Before the patient experiences a fracture at a significant bone location, such as the hip, spine, proximal humerus, pelvis, and/or wrist, with or without trauma, it remains asymptomatic until further diagnosis and treatment11, 12.
As the population ages, osteoporosis will become more prevalent and will have a greater influence on clinical, economic, and social outcomes for people of all sexes and ethnicities11, 13. The treatment cost of a hip fracture in developing countries, like Malaysia, is expected to increase from 35.3 million USD in 2018 to 125.4 million USD in 205013. Increasing age, female sex, postmenopausal status, hypogonadism or premature ovarian failure, low body mass index, ethnic background, rheumatoid arthritis, low bone mineral density (BMD), vitamin D deficiency, low calcium intake, hyperkyphosis, smoking, alcohol abuse, immobilization, and long-term use of certain medications are a few of the factors that can cause osteoporosis14.
Antiresorptive and anabolic therapies have been established for the treatment of osteoporosis using numerous drugs and biomaterials with the aim of activating bone formation or suppressing OC function and survival15. To provide more applicable preclinical research results, scientists have concentrated on developing models of bone cells utilizing cell lines or primary cells16. Studies have been conducted on primary cells, such as primary mouse mesenchymal progenitor cell-derived OBs17, 18, primary human osteoblast (Hob) cells, and PMBC-derived OCs19. Cell lines include the human fetal osteoblast cell line (hFOB 1.19)20, RAW264.7-derived OCs21, and MC3T3-E1 OBs22. Because OBs and OCs are important during osteogenesis and remodeling, these cells are used for studies of bone diseases23, 24. These cells do not behave independently of one another, and various communication pathways between them have been discovered24.
Therefore, the complex interactions of OBs and OCs and their precursors during bone remodeling are best studied and understood using co-culture, which may provide more information on the engineering of bone tissues25. The monoculture model involves only one cell type in a culture medium, whereas the co-culture method includes multiple cell types, which are cultured together in the same medium. This review summarizes the effects of polyphenols on both OBs and OCs using a monoculture model. Further research on polyphenols should be conducted with the development of a co-culture model as it allows for optimal cell–cell interactions and mimics the environment more accurately than a monoculture26.
Polyphenols
The class of phytochemicals known as polyphenols is present in a variety of plants, including apples, berries, citrus fruit, plums, broccoli, chocolate, tea, and coffees27, 28. Phenols (hydroxybenzenes), especially polyphenols (containing two or more phenol groups), are synthesized by plants; they perform important roles under certain difficult conditions, such as when pathogens are present or when the climate is challenging28, 29. Polyphenols can be divided into four significant groups determined by the number and binding structure of the phenol units: flavonoids, stilbenes, lignans, and phenolic acids (Figure 1)30, 31.
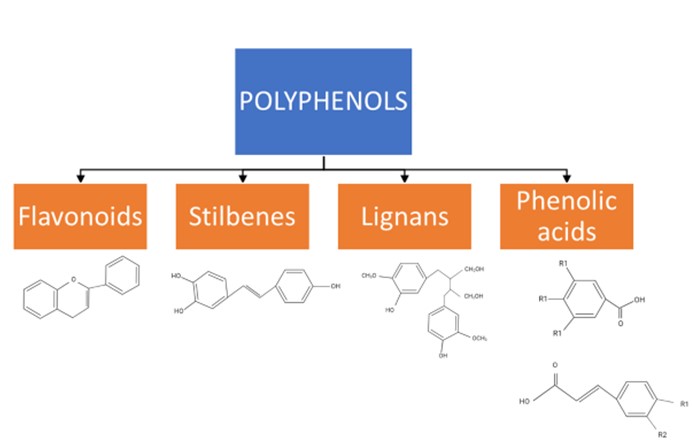
Polyphenols group and chemical structures of the different groups of polyphenols.
Flavonoids
Flavonoids can be found in edible plants, such as cranberries () and apples (). They are present as O-glycosides with sugars, such as glucose and/or rhamnose, linked to the phenolic hydroxyl groups or directly bonded to the carbon skeleton32. Flavonoids can also exist as polymers or aglycons in the seeds of plants32. Anthocyanins, flavanols, flavanones, flavones, flavonols and isoflavonoids are the main classes of flavonoids33.
Effects of polyphenols on OBs and OCs
Polyphenols |
Bioactive compound |
Effects on OBs |
Effects on OCs |
---|---|---|---|
Flavonoids |
(2S,3S)-Aromadendrin-6-C-β-D-glucopyranoside (AG) from the extract of U. wallichiana (Himalayan Elm). |
Swarnkar - increased differentiation of preOBs obtained from neonatal mouse calvaria. - elevated gene expression of osteogenic markers, Runx2, Bmp2, Col1, and Bglap in preOBs. - increased extracellular matrix mineralization in preOBs and bone marrow cells. - protected the differentiated OBs from serum deprivation-induced apoptosis. |
Swarnkar - increased the expression of the anti-osteoclastogenic cytokine, Tnfrsf11b. - inhibit OCs differentiation of bone marrow precursor cells to OCs in the presence of RANKL and M-CSF. |
Kaempferol [3,5,7-trihydroxy-2-(4-hydroxyphenyl)-4 H-1- benzopyran-4-one] |
Tsuchiya - increased Alpl activity and calcium deposition. - increase Runx2, Bglap, Sparc, Spp1 Guo - activated the transcriptional activity of pERE-Luc and induced estrogen receptor α (ERα) phosphorylation that was correlated with induction and associated with OBs differentiation biomarkers. - promoted the mineralization process of OBs. |
Kim - reduced TRAP-positive cells and resorption pits. - reduced RANKL, TRAF6, c-Fos, NFATc1. - reduced p-ERK and p-JNK. - reduced beclin-1 and SQSTM1/p62 Wattel - reduced bone resorption in dose and time dependent manner. - directly induced apoptosis of mature OCs in same dose-range effective for inhibiting bone resorption. | |
Luteolin (3',4',5,7-tetrahydroxyflavone) |
Melguizo-Rodríguez - elevate the expression of Runx2, Alpl, Col1, Sp7 and Bglap, Choi, (2007): - increased collagen content, ALP activity, and |
Crasto - produced deeper resorption pits, but with decreased surface area, resulting in overall decreased pit volume. - disruption of OCs V-ATPase | |
Stilbenes |
Piceatannol (3,3',4,5'-tetrahydroxy-trans-stilbene) |
Chang - increased alkaline phosphatase activity and mRNA expression in a dose-dependent manner. - increased the levels of Bglap protein. - increased Col1 protein levels. - increased the amount of OBs mineralization in a dose-dependent manner. |
Yan - attenuated RANKL-induced OC differentiation. - inhibited OC-mediated bone resorption. - inhibited RANKL-stimulated OC-specific gene expression. - suppressed RANKL-stimulated activation of NF-kB, JNK, ERK and AKT. - promoted caspase 3-mediated apoptosis of mature OCs. |
Lignan |
Gastrodin |
Liu - increased mRNA levels of osteogenic genes (Runx2, OSX, Bmp2 and Bglap). - increased Alpl activity and calcium deposit |
Zhou - inhibited RANKL-induced OCs differentiation by downregulating the expression of NFATc1. - inhibited gene expression of Dcstamp thus preventing OCs maturation and migration. - prevented RANKL induced-osteoclastic bone erosion. |
Phenolic acid |
Tannic acid |
Hapidin - increased proliferation by increasing OBs cell number. - OBs morphology was uniformly fusiform shaped with filopodia extensions. - improved mineralization by increasing the percentage of Ca and P. |
Steffi - reduced TRAP activity and OCs cell number. |
3‐(3‐hydroxyphenyl) propionic acid |
Chen - stimulated OBs cell differentiation. - increased OBs cell differentiation markers ( |
Zhao - inhibited osteoclastogenesis through a RANKL‐RANK independent mechanism. - reduced osteoclastogenesis and OCs resorptive activity in dose-dependent manner. - inhibited NFATc1 expression, with a subsequent reduction in expression of downstream osteoclastogenic marker genes. |
(2S,3S)-aromadendrin-6-C-ß-D-glucopyranoside (AG) is a flavonoid isolated from the stembark of 34. Swarnkar . (2011) found that AG treatment significantly increased the differentiation and expression of mineralization markers on OBs isolated from the calvaria of 1–2-day-old Balb/c mice. AG treatment for 21 days produced a 40% increase in mineralization (Alizarin red staining) compared with a control at 100 nM (10M)34. In addition, 48-h treatment with AG increased alkaline phosphatase (Alpl) production by 80% over control at 100 nM34. Furthermore, 24-h treatment increased mRNA levels of runt-related transcription factor 2 (), and 48-h treatment increased bone morphogenetic protein 2 (), collagen type-1 (), and osteocalcin (), which are important osteogenic gene expression markers34.
In addition to the increase in OB differentiation, AG subsequently increased osteoprotegerin (Tnfrsf11b) levels after 24 h and 48 h of treatment34. Tnfrsf11b is a soluble decoy receptor for the nuclear factor-B ligand receptor activator, which is essential for the differentiation of OCs35. In contrast, AG directly inhibited OC differentiation by blocking RANKL+ macrophage colony stimulating factor 1 (M-CSF-1)-induced osteoclastogenesis in murine bone marrow cells, as evidenced by reduced expression of OC phenotypic markers. In addition, treatment with 100 nM AG on day 6 decreased mRNA levels of tartrate-resistant acid phosphatase (TRAP), c-fos, RANK, and cathepsin K ()34.
Kaempferol [3,5,7-trihydroxy-2-(4-hydroxyphenyl)-4 H-1-benzopyran-4-one] is a natural flavonoid with a low molecular weight that is present in foods like broccoli, cabbage, beans, tomatoes, strawberries, grapes, and tea36. Previous studies have shown that kaempferol exhibits antioxidant and anticancer activities and 36. Furthermore, it can be applied to the management of osteoporosis. Wattel . (2003) reported that kaempferol significantly reduced bone resorption by promoting spontaneous OC apoptosis37. OCs were obtained and purified from unfractionated bone cells from long bones of 10-day-old rabbits and were cultured for 48 h before treatment with kaempferol38. Treatment with 50 µM kaempferol increased the number of apoptotic OCs compared with a control. Another study on murine macrophage RAW264.7 cells treated with RANKL for 9 days reported that treatment with 50 µM kaempferol inhibited RANKL-induced differentiation of RAW 264.7 cells39. In addition, the treatment inhibited the activation of extracellular signal-regulated kinase (ERK) and c-Jun N-terminal kinase (JNK) of the mitogen-activated protein kinase (MAPK) pathway, which subsequently reduced the expression of RANKL, TNF receptor-associated factor 6 (TRAF6), c-Fos, and NFATc1. Furthermore, kaempferol treatment suppressed OC autophagy-related proteins, such as beclin-1 and SQSTM1/p6239.
Kaempferol-immobilized titanium dioxide (TiO) increased osteogenic activity in rat bone marrow stromal cells (rBMSCs) isolated from femurs of 6-week-old female Sprague–Dawley rat femurs40. TiO is usually used for endosseous implant materials, and two samples of alkali-treated TiO were evaluated in this study: the coprecipitation sample (Al-cK), immersed in DPBS containing 50 μg kaempferol/100% ethanol, and the adsorption sample (Al-aK), in which 50 μg kaempferol/100% ethanol was dropped onto control samples40. The results showed that the calcium deposition of rBMSCs after 7 days on Al-aK was significantly higher than in the control, Al-Ti, and Al-cK samples40. In addition, calcium deposition in both the Al-aK and Al-cK samples was significantly higher than in the control and Al-Ti sample after 14 days of treatment40. Furthermore, the mRNA expression of OB-related proteins, such as osteocalcin (Bglap), osteonectin (Sparc), osteopontin (Spp1), and Alpl in rBMSCs grown on Al-aK and Al-cK was higher than in rBMSCs grown on the control and Al-Ti on day 3 and 740.
Another study found that kaempferol stimulated the osteogenic differentiation of cultured OBs by acting through estrogen receptor (ER) signaling evidenced by the induction effect on pERE-Luc-transfected cultured OBs41. Primary rat OBs were obtained from calvarial bones from 2-day-old neonatal Sprague–Dawley rats. OBs was cultured for 21 days and treated with kaempferol (10 µM) or 17β-estradiol (100 nM) in the presence of β-glycerophosphate (20 ng/mL) at 3-day intervals41. In OBs expressing pERE-Luc, 50 µM kaempferol and 17β-estradiol increased luciferase activity in a dose-dependent manner41. Furthermore, 30 µM kaempferol and 17β-estradiol increased Alpl activity and osteoblastic mineralization41. However, pre-treatment with ICI 182,780 (estrogen receptor inhibitor) fully blocked kaempferol-induced and 17β-estradiol pERE-Luc activity and Alpl activity, indicating that kaempferol acts via ER activation41. In addition, kaempferol and 17β-estradiol treatment of OBs significantly increased the transcription of numerous genes of bone differentiation markers, such as , , , , and ; this transcription was also blocked by pre-treatment of ICI 182,78041.
Another naturally occurring flavonoid is luteolin (3',4',5,7-tetrahydroxyflavone), which typically appears in glycosylated forms in celery, green pepper, perilla leaf, and chamomile tea and as an aglycone in perilla seeds42. A study on the MG63 OB cell line reported that 10M luteolin treatment for 24 h enhanced OB-related gene expression, including expression of , , , , and 43. Luteolin also has an anabolic effect on osteoblastic MC3T3-E1 cells through an estrogen-mediated mechanism21. For example, luteolin significantly enhanced collagen production in osteoblastic MC3T3-E1 cells (at 1 µM), Alpl activity (at 0.1 and 1 µM), and Bglap secretion (at 1 and 10 µM) after 7 days of culture21. However, the effects of luteolin on increasing collagen synthesis and Alpl activity were inhibited by the anti-estrogen drug tamoxifen, indicating that luteolin is involved partly in the mechanism of estrogen action in osteoblastic cells21.
Crasto . (2013) conducted a study on OCs differentiated from murine macrophage RAW 264.7 cells from ATCC and bone marrow mononuclear (BMM) cells isolated from tibias and femurs of 6-week-old CD-1 (5 days of RANKL and M-CSF stimulation with or without luteolin). The EC for both cells was 1.2 mM and 2.5 mM respectively. Luteolin treatment inhibited bone resorption via disruption of OC V-ATPase44. V-ATPases are recruited to the plasma membranes of polarized, active OCs during bone resorption, where they regulate extracellular acidification44. Furthermore, luteolin decreased the surface area of the resorption pit, which reduced the overall volume of the pit while inhibiting OC bone resorption without altering OC actin ring formation44.
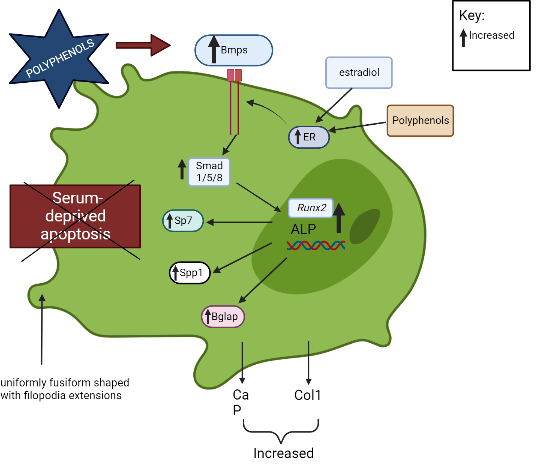
Schematic representation of the potential effects of polyphenols on osteoblast (OBs). Polyphenols upregulated bone morphogenetic protein-2 (Bmp2) and activate Smad proteins through a complex serine threonine receptor mechanism which subsequently induce the differentiation of bone marrow stem cells into OBs and modulate the expression of OBs related genes such as the runt related transcription factor 2 (Runx2) and alkaline phosphatase (Alpl)
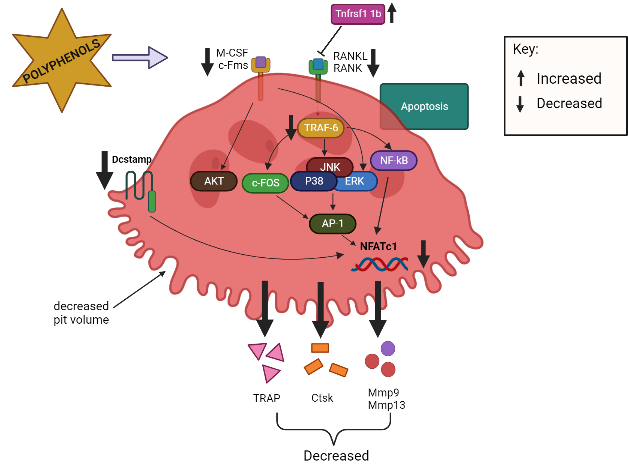
Schematic representation of potential effects of polyphenols on osteoclasts (OCs). Receptor activator of nuclear factor-κB ligand (RANKL) binds with RANK on preOCs, TNF receptor-associated factor (TRAF) 6 is recruited, which leads to activation of various transcription factors such as mitogen activated protein kinases (MAPK) including c-Jun N-terminal kinase (JNK), extracellular signal-regulated kinase (ERK), and p38, c-Fos, nuclear factor kappa B (NF-κB), and nuclear factor of activated T-cell (NFATc1)
Stilbenes
Flavonoids are the most prevalent phenolic chemicals found in food, whereas stilbenes are rarely found in the human diet54, 55, 56. Flavonoids can be found in plants, such as grapevine, berries, and peanuts57. Two benzene rings connected by an isopropylene moiety to form a compact ring structure and separated by a double bond define the structure of stilbenes55.
Piceatannol (3,3',4,5'-tetrahydroxy-trans-stilbene), or PIC, is found in grapes, passion fruit, white tea, Japanese knotweed, Asian legume, and Korean rhubarb. PIC exhibits antioxidant, antitumor, and anti-inflammatory activities, without toxicity in humans58. Chang . (2006) reported that PIC stimulates MG-63 and hFOB (both are OB-like cell lines) maturation and differentiation. PIC treatment did not improve cell proliferation but significantly increased (48 h treatment) and (72 h treatment) protein and gene expression at 0.1 – 20 µM. PIC treatment also increased Col1 synthesis (72 h treatment) and mineralization (96 h treatment)59. In addition, PIC increased the expression of at both the transcriptional and translational level in a time- (6 – 24 h) and dose-dependent manner (1 – 2 µM). Therefore, PIC treatment can increase the differentiation of MG-63 and hFOB cells from the cell maturation stage of development to the matrix maturation stage59.
PIC also significantly affects OC differentiation and bone resorption51. OCs were differentiated from RAW264.7 cells via RANKL and M-CSF stimulation. PIC significantly reduced TRAP-positive OCs and inhibited OC development in a dose-dependent manner (0, 2.5, 5, 10, 20, 40 mM) after 4 days of treatment51. The treatment also significantly reduced bone resorption pits, increased mature OC apoptosis, and decreased mature OC survival in a dose-dependent manner51. PIC treatment reduced the mRNA expression of NFATc1, dendritic cell-specific transmembrane protein (Dcstamp), Ctsk, matrix metallopeptidase-9 (Mmp9), and TRAP induced by RANKL. In addition, PIC treatment inhibited the phosphorylation of osteoclastic genes, including JNK, ERK1/2, nuclear factor kappa B (NF-κB), p65, IκB kinase alpha (IκBα), and serine/threonine kinase (AKT)51.
Lignans
Lignans are a class of diphenolic compounds that are produced when two phenylpropanoid C6-C3 units are combined at the β and β’ carbon and can be further linked by ether, lactone, or carbon bonds60. However, when the molecular linkage of monomers occurs the other way around, the compound is classified as a “neolignane”61. Lignan is found in plants, such as flaxseed, sesame, and seeds of 62.
Gastrodin (GSTD), also known as gastrodia glycoside, is a phenolic compound that is found in a well-known Chinese medicine63. It has been used for many years as an anticonvulsant, analgesic, and sedative agent against vertigo, general paralysis, epilepsy, and tetanus63. Liu (2018) reported that this compound has potential in improving osteoporosis. Their study was conducted on dexamethasone (DEX)-induced cellular dysfunction of MC3T3-E1 OBs. GSTD (≤ 100 µM) 2 h pre-treatment was able to maintain the cell viability of MC3T3-E1 OBs at high concentrations of DEX (≥ 50 µM) following 24 h of exposure22. Furthermore, 7 days of 1–5 µM GSTD treatment significantly increased Alpl activity, which was reduced by DEX22. In addition, via the NRF2 signaling pathway, GSTD promoted osteogenesis and maintained the balance between adipogenesis22. Treatment enhanced the expression of bone osteogenic markers, such as , , , and 22. In addition, GSTD treatment enhanced the formation of calcium nodules, upregulating OB osteogenic differentiation and enhancing the maturation process of MC3T3-E1 cells22. Pre-treatment with GSTD for 1 h also significantly reduced DEX-induced apoptosis of OBs22.
An study conducted by Zhou . (2017) reported that GSTD suppressed osteoclastogenesis by downregulating the nuclear factor-activated T cells c1 (NFATc1) signaling pathway while promoting osteointegration33. OCs were derived from BMM cells via RANKL and M-CSF stimulation. GSTD (2 and 10 µM) suppressed RANKL-induced OC differentiation in the early stage of culture (day 0–2) in a dose-dependent manner and attenuated OC differentiation at the terminal stage of culture by inhibiting the migration of OCs to resorb into the bone slice22. Moreover, GSTD reduced the expression of NFATc1 in BMM cells on days 1 – 3, which subsequently reduced the fusion and migration of pre-OCs by downregulating OC-specific gene expression, including the expression of and33.
Phenolic acids
Phenolic acid is another bioactive compound that exists in many plants. In its structure, hydrogen atoms on benzene rings are replaced by a carboxylic acid group with at least one hydroxyl64. Benzoic acids (gallic, p-hydroxybenzoic, vanillic, and syringic acid) and cinnamic acid are the two major phenolic chemicals from which phenolic acid is generated (caffeic, ferulic, sinapic, and p-coumaric acids)65.
Tannic acid (TA) is a naturally occurring polyphenol that is found in red wine, beer, coffee, black tea, green tea, grapes, pears, bananas, sorghum, black-eyed peas, lentils, and chocolate66. TA has been used as a food additive, a medication to treat diarrhea, a local astringent, an antidote for poisoning, and a remedy for burns67. It also possesses antioxidant, antimutagenic, and anticarcinogenic activities67. TA treatment is more effective in increasing hFOB 1.19 cell proliferation (EC= 2.94 M) than pamidronate (PAM) (EC= 15.27 M), a nitrogen-containing bisphosphonate that is used to inhibit bone resorption20. TA treatment also increased the calcium phosphate (Ca/P) molar ratio in a time-dependent manner (day 3 and day 10), which is crucial for the mineralization of the extracellular matrix20. Moreover, treatment of hFOB 1.19 cells with TA produced a confluent monolayer of cells, a significant number of bone nodules, and large globular accretions with flattened orientation20. Compared with cells treated with PAM, TA produced better results in terms of proliferation, morphological alterations, and mineralization20. In contrast, a study by Steffi . (2019) reported that TA treatment of RAW 264.7 cells reduced RANKL-stimulated TRAP activity on day 5 of culture. The treatment also reduced the OC number measured by total DNA on day 5 of culture68. Furthermore, the treatment reduced the actin ring formation of OCs68. The effects of phenolic acid on OBs and OCs are presented in
Following consumption of coffee, vegetables, blueberries, and other fruits, a polyphenol molecule called 3(3-hydroxyphenyl) propionic acid (PPA), a phenolic acid that is generated by the gut microbiota, is released into the bloodstream69, 70. The breakdown of chlorogenic acid by the gut microbiota results in the production of PPA, which is absorbed and oxidized in the liver before entering the circulation69. PPA treatment (1–100 µg/dL) increased Alpl production in bone marrow-derived mouse stromal cell line ST2 cells after 10 days of culture70. In addition, 24 h of treatment increased the mRNA expression of various OB differentiation markers, including Col1 and Spp170. Moreover, Zhao . (2020) reported that PPA can suppress osteoclastogenesis through the RANKL‐RANK independent pathway in RAW 264.7 cells and nonadherent bone marrow cells isolated from 4‐week‐old female C57BL/J mice. PPA treatment (1 – 100 µg/dL) with RANKL and M-CSF has been shown to decrease the number of OCs and bone resorption pits per well69. In addition, PPA treatment for 3 days inhibited RANKL-induced NFATc1, cFos, Mmp9, and Ctsk protein expression. PPA reduced the expression of the second messenger GPR109A on the surface of pre-OCs, which increased the level of cAMP inside cells and inhibited the expression of OC-specific genes and OC development69.
Possible molecular mechanisms involved on the effects of polyphenols on OBs and OCs
The BMP signal transduction pathway regulates OB formation and activation via both conventional Smad-dependent (Bmp ligands, receptors, and Smads) and non-canonical Smad-independent signaling pathways (p38 MAPK pathway)45. A previous study demonstrated that polyphenols promoted OB activation and development via the Smad-dependent signaling pathway45, 46. Polyphenols upregulate and activate Smad proteins through a complex serine threonine receptor mechanism that subsequently induces the differentiation of bone marrow stem cells into OBs and modulates the expression of OB-related genes (, )45, 46.
is a member of the runt family of transcription factors 1 and is crucial to several stages of bone development71. The roles of include the establishment of the lineage of OBs from multipotent mesenchymal cells, promotion of early OB differentiation, and inhibition of late OB differentiation72. regulates the expression of , which is required for OB differentiation and bone formation, by directly binding to the promoter47, 48. is also involved in the OB-selective expression of BSP when interaction occurs between two types of enhancers: a homeodomain protein-binding site (the C site) and two Runx2-binding sites, R1 and R273. BSP belong to the “small integrin-binding ligand N-linked glycoproteins” (SIBLING), an extracellular matrix protein family of mineralized tissues that is involved in the initial steps of bone mineralization74. is another example of an OB-specific gene that is influenced by 75, 76. is crucial in the bone remodeling process, and a study found that due to the manipulation of the OC lacunar-canalicular network remodeling in the cortical bone, -knockout mice had decreased resistance to fractures in their long bones, indicating that Mmp13 is required for the proper distribution of mineral density in cortical bone77. Furthermore, downregulates the expression of by binding to the promoter at -252 bp and -84 bp, which subsequently promotes the osteoblastic differentiation of primary mesenchymal progenitor cells17. determines the OB lineage from pluripotent mesenchymal cells, enhances OB differentiation at an early stage, and inhibits OB differentiation at a late stage.
Polyphenols increase the production of calcium, phosphorus, and Col1, which indicates OB mineralization and bone growth49. Some polyphenols, like (2S,3S)-aromadendrin-6-C-β-D-glucopyranoside (AG) from the extract of , can protect the differentiated OBs from serum deprivation-induced apoptosis34. In addition, polyphenols stimulate OB formation and mineralization through the ER. Estrogen-induced messengers are effectively transmitted in OBs by estrogen receptor alpha (ERα)78. Through ER signaling, polyphenols, like kaempferol, induce osteogenic differentiation of cultured OBs, which subsequently increases the transcription of numerous genes of bone differentiation markers (, , , , and )41.
OC precursors differentiate into mature OCs primarily through interactions with two cytokines: M-CSF and RANKL79. OC precursor cells require signals for proliferation and survival from M-CSF, which binds to colony-stimulating factor 1 receptor (c-Fms), while RANKL-to-RANK interactions are important for differentiation, resorptive activity, and the survival of mature OCs52. When RANKL binds to RANK on pre-OCs, TRAF6 is recruited, which activates various transcription factors, such as MAPKs (JNK, ERK, p38), c-Fos, NF-κB, and NFATc139, 50, 80. c-Fos is essential for the activation of activator protein-1 (AP-1), which interacts with NFATc181. This interaction regulates the expression of genes necessary for OC differentiation and subsequently regulates several OC-related genes, including , , , and 50. Polyphenols can reduce the RANKL-induced differentiation of OCs by inhibiting the expression of mRNAs related to OC differentiation, including TRAP, , , , and in primary osteoclastic cells33, 51, 82. Tnfrsf11b, a soluble RANKL decoy receptor that is primarily generated by OBs, inhibits the interaction between RANKL and RANKL receptors, thereby hindering the development of OCs and inhibiting osteoclastic bone resorption35. Swarnkar . (2011) found that polyphenols increased the production Tnfrsf11b, which subsequently inhibited OC differentiation.
On the other hand, the binding of M-CSF to c-Fms receptor results in increased OC precursor proliferation and survival through the ERK and PI3K/AKT pathways52. Polyphenols inhibit the differentiation of bone marrow precursor cells to OCs in the presence of RANKL and M-CSF and suppress the activation of AKT34, 51. Dcstamp is a multi-pass transmembrane protein, another master regulator of osteoclastogenesis that is essential for the cell–cell fusion of OC precursors during OC development83, 53. Dcstamp possibly interacts with NFATc1 to ensure successful OC differentiation53. Zhou . (2017) reported that polyphenols can inhibit the gene expression of Dcstamp, thereby preventing OC maturation and migration33. Figure 2 and Figure 3 summarize the targeted pathways involved in bone formation and the suppression of OC function and survival.
Co-culture
According to Sieberath. (2020), researchers have focused on the development of models using cell lines or primary cells to obtain more relevant preclinical results related to bone cell studies. This is due to the limitations and ethical issues faced when using animal models; therefore, if possible, they should be replaced with studies16. Co-culture provides a novel approach for the biological study of biomaterials, as the cellular environment in co-culture is closer to the environment than monoculture, with appropriate cell–cell interactions26. The co-culture model can be established both in 2D and 3D arrangement, with or without a direct physical contact among different cell types, and static or dynamic systems (Figure 4)84.
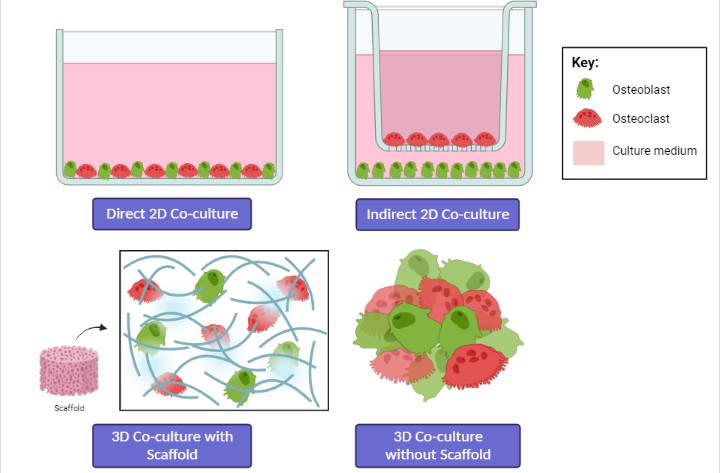
Various technique of co-culture in in vitro model of bone remodeling process.
Direct or indirect co-culture: advantages and limitations
Co-culture methods involve the cultivation of two or more different cell types and can be performed in one culture dish or well either directly or indirectly85. In direct co-culture systems, cells are mixed in the culture environment and can make direct contact with each other86. Direct co-culture can be performed in almost any cell culture dish, for example, by layering two cell types on top of each other. In contrast, indirect co-culture is performed by separating different cell types using inserted porous membranes85. Cells in direct co-culture can connect with each other in various ways, such as through gap junctions, tight junctions, and desmosomes86. Direct cell–cell contact is reported to play an important role in various mechanisms, and direct co-culture methods allow physical interactions and the analysis of autocrine/paracrine signals84, 85. Therefore, the direct co-culture system mimics conditions24. However, the inability to distinguish the different contributions of the diverse cell types, as the cells are mixed together in the same environment, is a clear disadvantage of direct co-culture84. Despite its simple set-ups, this methodology is associated with numerous technical difficulties, whereas indirect co-culture takes advantage of cell cultures inserted with porous membranes to keep the co-cultivated cell populations separate24, 26. For example, in direct co-culture, the growth dominance of OBs causes the massive death of OCs, which is unfavorable for long-term culture87. Therefore, cell ratios must be optimized24. In addition, it is difficult to isolate a single type of cell from the co-culture system, which limits the methods available to analyze cells separately24, 87.
In indirect co-cultures, cells are physically separated; however, culture medium and other molecules, such as proteins, extracellular vesicles, and soluble factors, released by one cell type can still cross the pores and influence the behavior, proliferation, maturation, and differentiation of the other cell type(s) through paracrine signaling84. Moreover, cells can be evaluated separately, and cell migration can also be analyzed24. The limitation is that the physical receptor-mediated cell–cell interactions are hindered, and the large volumes of cells needed might limit the oxygen supply in the bottom wells24, 84. In contrast, direct co-culture allows for a uniform medium height and oxygen supply for both cell types, and smaller volumes of cells are needed in direct co-culture than in transwell co-culture24.
Conclusion
Osteoporosis is becoming a major public health problem, and its incidence is increasing. Consequently, antiresorptive and anabolic therapies have been developed for the treatment of osteoporosis using various drugs and biomaterials with the purpose of stimulating bone formation or suppressing OC function and survival. According to previous studies, polyphenols, especially bioactive phenolics, have positive effects on bone metabolism in osteoporosis. OBs and OCs are the most conventional cell types for studying bone diseases as these cells are the crucial components of osteogenesis and remodeling.These cells do not behave independently of one another, and various communication pathways between them have been discovered. Therefore, it is imperative to further investigate the effects of polyphenols in co-culture models, as these models allow for optimal cell–cell interactions and mimic the environment more accurately than monoculture.
Abbreviations
Alpl: alkaline phosphatase, AKT: serine/threonine kinase, AP-1: activator protein-1, Bglap: osteocalcin, BMP: bone morphogenetic protein, c-Fms: colony-stimulating factor 1 receptor, Col1: collagen type 1, Ctsk: Cathepsin K, Dcstamp: dendritic cell-specific transmembrane protein, ERK: extracellular signal-regulated kinase, JNK: c-Jun N-terminal kinase, M-CSF: macrophage colony stimulating factor 1, NFATc1: nuclear factor-activated T cells c1, NF-κB: nuclear factor kappa B, Sp7: osterix, Sparc: osteonectin, Spp1: osteopontin, RANKL: receptor activator of nuclear factor-κB ligand, RUNX2: runt related transcription factor 2, Tnfrsf11b: osteoprotegerin, TRAP: tartrate-resistant acid phosphatase
Acknowledgments
The authors were grateful to the Ministry of Education, Malaysia for funding the publication cost of this work under the Fundamental Research Grant Scheme (FRGS) grant number FRGS/1/2020/STG01/USM/02/16.
Author’s contributions
Hermizi Hapidin developed the idea for the study and coordinated the article; Nurul Husna Azizul collected most of the provided data and drafted the article; Hasmah Abdullah, Maryam Azlan, Azlina Ahmad, and Ima Nirwana Soleiman participated in the critical revision of the article; and all authors read and approved the final version of the article.
Funding
Ministry of Education, Malaysia has funded the publication cost of this work under the Fundamental Research Grant Scheme (FRGS) grant number FRGS/1/2020/STG01/USM/02/16.
Availability of data and materials
Not applicable.
Ethics approval and consent to participate
Not applicable.
Consent for publication
Not applicable.
Competing interests
The authors declare that they have no competing interests.