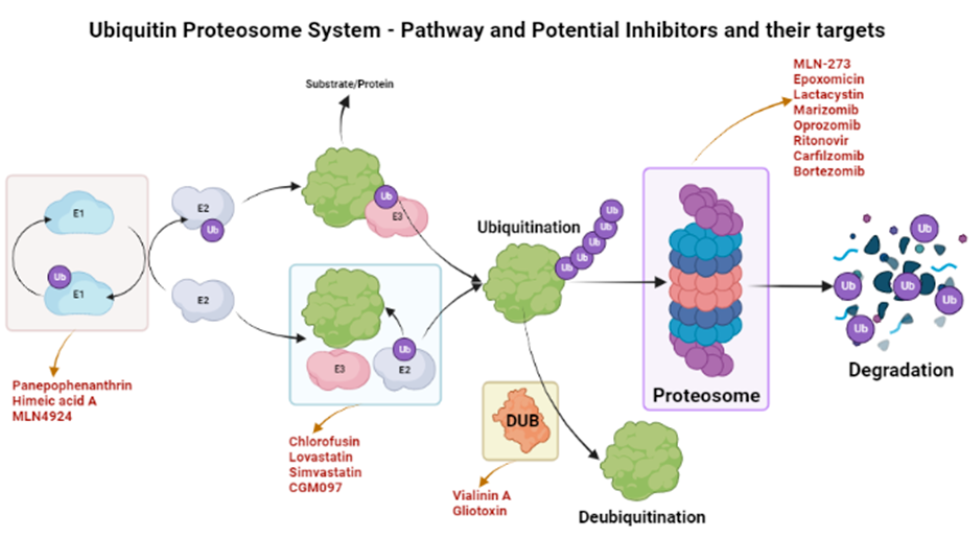
Therapeutic Potential of Microbial Metabolites: New Insights and Perspectives
- Molecular Medicine and Cancer Therapeutics Lab, Department of Zoology, Faculty of Science and Technology, University of Central Punjab, Lahore, Pakistan
- Applied Molecular Biology & Biomedicine Lab, Department of Zoology, University of Narowal, Narowal, Pakistan
- Cell and Molecular Biology Lab, Institute of Zoology, University of the Punjab, Lahore, Pakistan
- Department of Structural and Cell Biology, Tulane University, USA
- Department of Zoology, University of Education, Lahore, Pakistan
- Department of Zoology, University of Okara, Punjab, Pakistan
- Department of wildlife & Ecology, University of Okara, Okara, Pakistan
Abstract
Microbes release important metabolites that regulate various physiological activities inside and outside of organisms. The human gastrointestinal tract is a reservoir of microbes that play important regulatory roles in modulating the immune system and numerous other physiological functions. Thus, there is substantial interest in these microbial products and their clinical significance. These microbial metabolites have shown promise as therapies for cancer, inflammation, neurological disorders, and many other diseases. Here, we discuss microbial metabolites with substantial therapeutic potential, including proteasome inhibitors, therapeutic enzymes, bacteriocins, polyamines, and flavonoids.
Introduction
Therapies involving metabolites produced and released by microbes have received substantial attention from the scientific and medicinal community in recent decades. Microbial metabolites are microorganism-produced compounds with potential therapeutic applications. Microbial metabolites are being assessed as novel therapeutic tools for various diseases, including cancer and immune disorders1. The first therapeutic use of microbes to treat infection occurred during World War II after Alexander Fleming isolated penicillin in 19282. Currently, researchers are using different biological and chemical methods to study the biological effects of microbial metabolites on humans3. There is a growing demand for using substances generated from microorganisms in medicine, agriculture, the food industry, and scientific research4. Developing anticancer drugs with reduced side effects from the microbiome has been a research priority for many years. Researchers have discovered that natural products can help treat cancer, illness, infection, allergy, and many other diseases5, demonstrating that microorganisms are viable sources of therapeutics. Microbes associated with the human body impact pathophysiological processes, including metabolic disorders, mental disorders, and even cancer6. produce 0.1% of known microbial secondary metabolites, produce 7.0% and other bacteria produce 1–2%7. Other diseases, such as tuberculosis (TB), have long been treated using natural remedies derived from microbial secondary metabolites8. Currently, four drugs, isoniazid, rifampin, pyrazinamide, and streptomycin, are used to treat TB. The World Health Organization (WHO) plan for TB helps patients limit its spread worldwide. Microbial natural products benefit patients, avoiding injections and acting as alternatives to synthetic drugs and other regular therapies9. Microbial amino acids have been utilized in nutritional supplements and food for humans and animals. It is promising and economically advantageous to produce essential amino acids on an industrial scale using microbial metabolites10. Piericidins are a large class of microbial metabolites commonly formed by species of the genus and comprise a 4-pyridinol core skeleton with a methylated polyketide side chain11. Piericidin application has been developed over time. broth culture was screened in 1993 to assess the antitumor effects of piericidin as a novel phosphatidylinositol turnover inhibitor. Piericidins have been isolated from soil, water, and insect samples12. Here we summarize the main types of microbial metabolites that exhibit different therapeutic properties and can be potentially of clinical and therapeutic use. These metabolites are either secreted by microbes outside of the body or associated with the microbiome.
Biomimicry of Microbial Metabolites
The word biomimicry is derived from the Greek meaning life, and meaning imitation. Biomimicry approaches are frequently used in drug discovery. The novel approach of microbial metabolite imitation can increase the chemical repertoire of future pharmaceuticals. In medicine, biomimicry involves the development of homologs of host-endogenous molecules that target specific receptors and provide a desirable result13. The future development of pharmaceuticals will likely be broadened by the use of microbial metabolites that imitate promiscuous ligand–receptor interactions. Xenobiotic nuclear receptors, such as PXR and AhR, are prototypical host receptors with weak ligand interactions. Microbial metabolite mimicry using PXR and AhR as model xenobiotic receptors has been found to result in powerful and non-toxic treatments, mediating pathophysiological disorders involving these receptors. It is also plausible that these weaker receptor-ligand interactions have evolved to the host's benefit to avoid receptor overstimulation, which may have certain negative effects. Additionally, not all microbial metabolites are advantageous; some promote inflammation and cancer development13. Microbial metabolite mimics with distinct antibacterial effect can be tested against intestinal bacteria or in consortia-inoculated germ-free animals to explore diversity control as a method for host disease control. Thus, mimicry enables the diversification of the microbiome and the upkeep of host health homeostasis by increasing the metabolite repertoire14. Kaempferol, a natural flavanol, has anti-arthritis properties, among other pharmacological effects. Intraperitoneal (20 mg kg d) and intragastric (200 mg kg d) kaempferol delivery has been assessed for efficacy and mechanistic action in collagen-induced arthritis (CIA) mice. Kaempferol retained in the gastrointestinal tract diversified the microbiota. These findings support the idea that microbiome diversity contributes to the therapeutic effect. Kaempferol mimics with strong microbial remodeling capabilities can be used for arthritis therapy15. Intestinal microorganisms may produce indole/indole-3-propionic acid, which, when activated by PXR, downregulates the TLR4-NF-B inflammatory pathway in mice; an indole/indole-3-propionic acid small-molecule mimic of PXR enhance receptor activation. Unlike other PXR xenobiotics, the small-molecules FKK5 and FKK6 mimic the natural indole metabolites, avoiding toxic effects16.
The path toward using microbial metabolites as potential therapeutics
Humans have used numerous conventional drugs formulated from herbs, fungi, and synthetic chemistry as pharmaceuticals for centuries16. These products have effectively cured ailments such as cancer17. However, due to the limitations of past pharmaceuticals in curing and treating novel medical problems, drug and pharmaceutical development has grown exponentially in the past five decades, replacing older drugs to eliminate severe side effects and improve efficacy. Scientists and researchers have become more interested in microbes and their products, and the positive findings in clinical studies evaluating microbial products have led companies and agencies to opt for these strategies in novel drug development18. Many strategies, such as metal-based compounds from microbes, enzymes, and proteasomes, have been targeted for their clinical and therapeutic significance (
Microbial proteasome inhibitors
Proteosomes are the regulatory machinery that regulates the homeostatic conditions of the body by removing and degrading regulatory proteins. Many of these proteins play a substantial role in developing immunity against pathogens19, 20. A proteosome of the TB-causing bacteria was targeted by two small drugs, namely, MMV019838 and MMV687146. These drugs showed promise for curing TB and achieved better outcomes in tests21. Proteosomes are Ntn-hydrolases (N-terminal nucleophiles) that require ATP to cleave amide bonds22, 23. Regulatory proteins such as CDK inhibitors, cyclins, and tumor suppressors are extremely vulnerable to this machinery if demonstrating abnormalities in structure or function. Proteosome Inhibitors (PIs) target proteosomes and affect their functionality19. Biophysical parameters, such as the local concentration of proteins and their binding affinities, are critical for the PI efficacy24. PIs enhance immune responses and control the growth of cancerous cells by preventing proteosomes from removing regulatory proteins19. PI development was initiated approximately fifteen years ago25; due to their immunosuppressive effects, PIs have demonstrated substantial potential in the development of drugs for inflammation, carcinoma, immune disorders, and muscular dystrophies25, 26. PIs have demonstrated particular efficacy for hematological malignancies, multiple myeloma, and multiple cell lymphoma27.
The ubiquinone–proteosome system (UPS) is a major protein turnover regulator in mammalian cells and can be effectively targeted by PIs to cure malignancies (Figure 1)28. Fellutamides are UPS inhibitors produced by . and . in the gastrointestinal (GI) tract of a marine fish. Fellutamides are potently cytotoxic against a wide range of cancer cells, including sarcoma cells, fibroblasts, solid tumor cells, and human epidermoid carcinoma KB cells29. The mechanisms by which UPS functions within protein systems are shown in Figure 1.
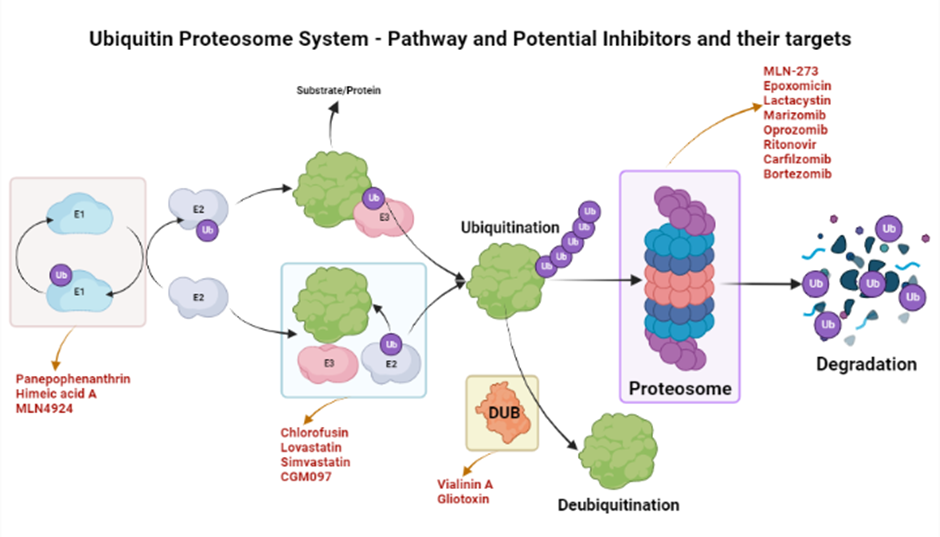
Overview of inhibitors of UPS. An overview of the action mechanism of the UPS. UPS is the protein degradation mechanism consisting of three important components i.e., ubiquitinating enzymes (E1, E2, & E3), a 26S Proteosome, and deubiquitinating enzymes (DUBs). These components can be specifically targeted by different inhibitors as shown in the figure. These inhibitors are in their respective phases of trials and some have been shown to be efficacious in different conditions such as inflammations, cancers, and anti-microbial activities.
Lactacystin, synthesized by 30, was the first-in-class PI discovered and incorporated into clinical research as a therapeutic31. Lactacystin effectively treats many diseases in animal models and is being evaluated in clinical trials. A rat model study used lactacystin to target UPS to address synaptic plasticity in Alzheimer’s disease (AD), successfully restoring synaptic tagging and capture (STC) and impairing activity-dependent synaptic plasticity and associative long-term memory . Thus, lactacystin is a potential therapy for AD32.
Belactosin (β-Lactone), mainly extracted from is a PI with antitumor properties and potential bioactivity against viruses and bacteria. Marizomib, a naturally occurring β-Lactone extracted from the , has shown clinical and preclinical improvements in multiple myeloma33, 34.
The clinical significance of various microbial metabolites against molecular targets suggests their promising role in clinical translation of novel drugs
Sr. |
Metabolite |
Microbial Specie |
Molecular targets |
Clinical Significance |
References |
---|---|---|---|---|---|
1 |
Prodigiosin |
Serratia marcescens |
Herpes simplex virus |
(PG) is a natural red pigment secondary metabolite. That exhibit cellular targets altering apoptosis and proapoptotic anticancer effects |
|
2 |
Serrawettin |
Serratia marcescens |
Methicillin-resistant Staphylococcus aureus (MRSA) |
Serrawettin, which was isolated from the green potato rhizosphere, has potent antifungal properties. According to reports, serratia produce antibacterial chemicals and secondary metabolites such as the red pigment prodigiosin. |
|
3 |
Ethyl acetate extract |
Pseudoalteromonas rubra, Virgibacillus salaries |
Nocardiopsis dassonvillei |
The ethyl acetate was used to extract the active compounds that were tested for bacterial growth inhibitory activity against human clinical pathogens. The EA extract was prepared as described and analysed for its content of triptolide and tripdiolide, which are responsible for up to 90% of the bioactiviA extract. |
|
4 |
Aminoglycosides (S-137-R) |
Bacillus velezensis |
Plasmid-mediated quinolone resistance |
Aminoglycosides (S-137-R) used for the treatment of severe Gram-negative bacterial infections. Streptococcal and enterococcal endocarditis can be treated with some treatments for severe Pseudomonas aeruginosa infections, brucellosis, and in low dosages as a synergistic approach. |
|
5 |
Methanolic pigment extract |
Micrococcus sp. |
Canthaxanthin (4′,4′-diketo-13-carotene) |
It is still being done to screen bioactive substances to uncover new chemical structures for the methanolic pigment extract utilized in pharmaceuticals. Some substances have been developed as antibiotics, and they are essential for the survival and growth of microorganisms in bacterial populations or the ability to withstand nutritional stressors. |
|
6 |
Germicidins, c-Actinorhodin |
Streptomyces lanatus |
Pyricularia oryzae |
Some Streptomyces strains produce germicidins, which serve as autoregulators of spore germination. |
|
7 |
Juglomycin A |
Streptomyces achromogenes E91CS4 |
Streptozotocin |
Juglomycins have bactericidal action against both Gram-positive and Gram-negative bacteria, as well as anticancer activity. There have been a few reports of racemic juglomycin syntheses |
|
8 |
Acyl depsipeptide (ADEP) |
Streptomyces hawaiiensis |
ClpP serine protease |
The caseinolytic protease (ClpP protease), the proteolytic centre of bacterial ATP-dependent proteases, was discovered to be the target of acyldepsipeptides (ADEPs), a new class of antibacterial chemical and its derivative. Treatment with ADEP lengthened Leptospira and slowed its growth kinetics. |
|
9 |
Lipopeptide lipid 430 |
Algibacter sp. M09B557 and M09B04 |
Chloropid, Bacteroidetes |
Human TLR2 transfected human embryonic kidney cells were triggered by Lipopeptide lipid 430, which also caused wild-type mice's blood CCL2 (MCP-1) levels to rise. |
|
Microbial therapeutic enzymes
Enzymes have long been used in industry and were initially explored in the context of medical therapy in the 1950s, altering our perception of medicinal drugs and therapeutics. Due to their anti-inflammatory and anticancer effects, enzymes have been developed for several ailments, including cancer, AD, and hyperuricemia44.
Inflammation is an immune response mostly characterized by swelling at the site of homeostatic disturbance due to environmental agents such as pathogens, chemicals, or abrasions45. Conventional drugs, such as non-steroidal anti-inflammatory drugs (NSAIDs), have been used to overcome inflammation; however, such drugs are associated with several side effects, such as GI ulcers. Trends have shifted toward the use of microbial enzymes to overcome these problems, for example, serratiopeptidase, which has high efficacy in inflammation and almost no harmful effects46. In a study on bowel disease in mice, the gut microbiota schistosome-derived enzyme P28GST (28 kDa glutathione S-transferase) showed promising anti-inflammatory results in the colon, restoring the regulatory responses between T-helper 1 and T-helper 2 cells47.
Serratiopeptidase is produced by the gram-negative bacterium . Serratiopeptidase is a protease in the trypsin family and demonstrates highly anti-inflammatory proteolytic activity48, 49. Serratiopeptidase restores wound sites through an unusual mode of action, recruiting immune cells from the lymph nodes to the affected area to promote healing50. The efficacy of serratiopeptidase increases exponentially when used in combination with other drugs, such as NSAIDs48.
Another protease enzyme, collagenase, hydrolyzes collagen fibers50. Collagenase was used in enzymatic wound debridement prior to the discovery of its anti-inflammatory properties. Das et al. assessed the anti-inflammatory application of collagenase in mice in 2018. Wound-healing macrophages loaded with collagenase santyl ointment (CSO) were implanted into the mice, and anti-inflammatory cytokine production was increased in the CSO-treated groups, improving inflammatory wound healing51. Similarly, the suppression of pro-inflammatory cytokines was observed when simvastatin-loaded porous microspheres were injected into the tendons of the collagenase-induced rats. The production of anti-inflammatory cytokines also increased52. Numerous other studies on the collagenase produced by have revealed that it is healthy, noninvasive, and safe in treating several pathological conditions53.
Superoxide dismutase is another clinically significant anti-inflammatory agent that can be derived from Cyanobacteria, such as , , , and 50.
L-asparaginase is a microbe-derived enzyme that can play an important role in the cure of cancer. Numerous molecules influence the proliferation of cancer cells and downregulate their growth50. L-asparaginase can be derived from , , , and 54, 55. L-asparaginase generates ammonia and aspartic acid by breaking down L-asparagine, which is the driver of its anti-cancerous ability. L-asparaginase is effective in treating lymphoblastic leukemia55.
Bacteriocins are the chemicals bacteria produce to eliminate competitors from ecological niches56 and are generally used in intra-specific interactions, for example, in competition for food and shelter57. Some bacteriocidins demonstrate anticancer activity with selective toxicity toward cancer cells58. Many bacteriocin molecules, such as Nisin A derived from are effective in head and neck squamous cell carcinoma (HNSCC) through inducing cell cycle arrest and blocking cell division59. Bacteriocins have also been found effective against breast cancer60. Bivocin H5C derived from , Laterosporulin 10 derived from , and Colicins A and E1 extracted from also have antitumor properties61.
Arginine deiminase is an enzyme produced and secreted by such as , and 61. Arginine has shown encouraging antitumor and anticancer properties and can play a key role in the cure and treatment of numerous auxotrophic carcinomas through arginine depletion62. The absence of arginosuccinate synthetase 1 (ASS1) in most cancers is the key aspect of the functionality of ADI (arginine deiminase). ASSI is critical for L-arginine production; cancer cells take up L-arginine for growth. This dependence of the cells on external sources can be pivotal in the anti-cancerous activity of arginine deiminases63.
Microbial antibiotic resistance is rising; thus, there the development of novel therapies is urgent64, 65. Antibacterial drugs and therapeutics are a prominent focus for handling this increasing challenge and controlling human and animal pathogens57. Several strategies and therapeutics have been developed, such as bacteriocins and lysin enzymes, referred to as enzybiotics. Enzybiotics are microbial enzymes and products that hinder the growth of pathogenic bacteria. Since their discovery in 2001, enzybiotics have played a significant role in the development of microbial based antibacterial drugs to combat antibiotic resistance44.
Bacteriocins are the secondary metabolites bacteria produce to target competitors, hindering the growth and reproduction of other microbes66. Bacteriocins are antimicrobial peptides (AMPs) 12–100 amino acids in length67. AMPs have recently been found effective against human microbial pathogens derived from lactic acid bacteria (LAB), 68. Bacteriocins have shown great efficacy towards several microbe-borne diseases, including methicillin-resistant (MRSA)69. LABs have been successfully employed in managing MRSA in clinical trials70. Another bacterial-produced TFnt, lysostaphin, is a bacteriocin that has demonstrated encouraging bactericidal activity against mutant strains71.
Endolysins are hydrolases that prevent the formation of the cell wall, cell membrane, envelope, and biofilm around antibiotic resistant strains of bacteria by lysing the peptidoglycan layer44, 72. Different endolysin enzymes have different mechanisms and target sites in Gram-positive and Gram-negative bacteria44. Many endolysins have shown efficacy in clinical trials. LysK-like endolysin derived from and LysSAP33 encoded by bacteriophage SAP33 have substantial lytic activity against the antibiotic-resistant targeting biofilm formation73. Similarly, many endolysins, namely, Abtn-4, derived from a bacteriophage D2 (vB_AbaP_D2), have shown substantial therapeutic potential, preventing biofilm formation by multiple phage-resistant Gram-positive and Gram-negative bacteria (, , , , and ). The aforementioned findings highlight the therapeutic potential of this endolysin74. Another important endolysin, LysAB54, was extracted from the phage p54 of the multi-drug resistant and showed promising antibacterial activity this and other gram-negative bacteria, such as and 75.
Other metabolites
Numerous other gut microbiome-derived metabolites also have immense therapeutic potential76. These metabolites play a substantial role in living organisms' biological machinery, influencing physiological, pathological, and metabolic activities and signaling pathways77. Gut microbial metabolites, such as short-chain fatty acids (SCFA) and many other organic compounds, have shown encouraging anticancer, brain modulation, anti-obesity, anti-bacterial, neurological, and mineral absorption properties78. These metabolites are very effective, especially for neurological diseases, including ASD, PD, AD, and neuroinflammation79, 80. Some other metabolites, such as TMA and TMAO, are also effective for cardiovascular diseases, including heart failure (72), hypertension81, and atherosclerosis82. Understanding the mechanisms of action of these metabolites will open doors for new therapeutic approaches81. Other such metabolites include TMAO, tryptophan, indoles, 4-ethylphenylesulfate, fatty acids, and protein-derived metabolites.
Protein-derived Metabolites
Protein-derived metabolites are functional, covalent protein modifications produced during or after protein digestion through endogenous, intrinsically reactive metabolites without the aid of enzymes83. Many protein-derived metabolites have been shown to be therapeutic. Taurine is a sulfur-containing β-amino acid and can be generated from cystine metabolism84, 85. Taurine has many important functions in animals' nervous system, muscles, kidneys, cardiovascular system, and immune system because of its anti-inflammatory and anti-oxidative effects85, 86. Taurine plays a vital role in maintaining homeostasis and many other physiological activities, such as gene expression and energy metabolism87, 88. Traumatic brain injury (TBI) is lethal, and inflammatory responses can result in death in other traumatic conditions. Taurine was administered to 32 patients with TBI conditions along with Standard Entera Meal. The levels of the inflammation biomarkers IL-6, IL-10, and TNF-α were recorded before and after the treatment, and serum IL-6 levels decreased significantly, showing the encouraging anti-inflammatory properties of taurine and its potential clinical use89. Taurolidine (TRD) is a taurine N-methylol derivative that exerts bactericidal and anti-inflammatory effects by inhibiting proinflammatory cytokines, such as IL-6, IL-8, and IL-1β90.
is another protein-derived microbial metabolite produced in the gut microbiome through histidine metabolism and is closely linked with glucose metabolism91, 92. disrupts glucose metabolism and causes several diseases, including type 2 diabetes and hypertension93, 94. T2D has been found to be closely associated with elevated , which alters histidine metabolism and impairs glucose metabolism and insulin signaling by activating Mtor1, p38γ, and S6K1 signaling92. is a potential activator of p38γ map kinase, inhibiting metformin activity95, and is associated with increased pro-inflammatory cytokines that cause gastrointestinal inflammation and inflammatory bowel disease93.
Microbiota-associated polyamines are organic cations produced by the gut microbiota organisms, such as 96. These polyamines are derived from arginine-containing proteins97 and are involved in numerous metabolic and cellular activities, including cell growth and differentiation and the production of DNA, RNA, and several proteins96. Many polyamines are also uremic toxins that hasten the progression of renal fibrosis and uremia98. However, polyamines such as spermine can suppress inflammatory cytokine production and regulate NF-κB activation96. Spermine is also associated with prostate cancer (PCa); significantly decreased levels of spermine have been observed in PCa patients. Spermine levels are a marker of PCa, and understanding the mechanism of action of spermine may lead to its use as a therapeutic target99. Another polyamine, putrescine, is naturally produced in peri-ovulating women and decreased in women with age-related infertility, demonstrating the role of putrescine in ova production and its potential for improving ova quality as a treatment for infertile women of advanced maternal age100.
Flavonoids
Flavonoids are plant-based nutritional constituents with substantial health benefits101. Certain flavonoids may have hepatoprotective characteristics that reduce the risk of coronary heart disease102. Moreover, these flavonoids have anti-inflammatory and anticancer properties103. Flavonoids demonstrate structural and functional effects through enzyme inhibition, acting as antioxidants, damaging cells, triggering host defense mechanisms, and blocking virus cell attachment and penetration103, 104. As secondary plant metabolites, flavonoids constitute the majority of the non-energetic aspect of human nutrition. Most dietary flavonoids are O-glycosides and are primarily consumed as D-glucose; fermented foodstuffs such as wine, tempeh, and certain teas also contain flavonoids. The polarity of the flavonoid molecule increases with glycosylation, which is necessary for preservation in plant cell vacuoles105. Similarly, many GI tract microbial metabolites may be responsible for or contribute to quercetin's effect when taken orally since the flavonoid metabolites DHPA and 4-methylcatechol reduce arterial blood pressure106. The absorption, distribution, digestion, and elimination of flavonoids by the GI tract are critical processes affecting human health107. These features are influenced by how flavonoids interact with other dietary components, the host, the environment, and GI flora108. Flavonoids can target the microbiome, various GI tract cell types, and compounds present in luminal material107, 108, 109.
Bile acids
Bile acids are microbiological byproducts with potential therapeutic applications. The gut microbiota produces bile acids, which are crucial for dietary lipid solubilization110. The gut microbiota facilitates digestion and has been linked to diseases and health risks. Despite the considerable variation in gut microbiota composition, it is challenging to link intestinal microbiota patterns to health and nutrition because the gut microbiota has a high level of functional redundancy. Metabolomics has successfully identified gut-produced microbial metabolites that may be significant mediators of diet-induced host–microbial interaction in several studies. Among the metabolites derived from nutrition are short-chain fatty acids, secondary bile acids inferred from primary bile acids, microbial tryptophan catabolites that originate from proteolysis, imidazole propionate originating from histidine, and trimethylamine N-oxide111, 112. Bile acids can potentially be used to treat non-alcoholic fatty liver disease (NAFLD), cholestatic and metabolic liver diseases, infant jaundice, and other liver problems113, 114, 115, 116. NAFLD is the most frequent chronic liver disease worldwide115. The link between the gut microbiota & NAFLD has been widely researched. The gut microbiota controls NAFLD by fermenting undigested foodstuff, interacting with intestinal mucosal immune system, and altering intestinal barrier function, leading to signaling changes117. Microbial metabolites, including SCFAs, TMAO, BAs, endogenous ethanol, and indole, play important roles in NAFLD regulation. However, changes in microbial metabolites in NAFLD are undeniable. Microbial metabolites impact the signaling pathway in the stomach and the liver, which is distant from the gut117, 118. Microbes, in part, control the host's immune system by generating metabolites. An increasing body of research suggests that some microbial metabolites affect the immune system significantly through host receptors and other target molecules. P2X7, GPR41, GPR43, GPR109A, aryl hydrocarbon receptor precursor (AhR), farnesoid X receptor (FXR), PXR, and TGR5 are some of the metabolite-specific receptors expressed by immune cells119, 120, 121. Changes in food, bodily functions, and the immune system produce a range of signals from microbial metabolites and their receptors. Gut bacteria produce many lipid-modifying and metabolizing enzymes. For instance, polyunsaturated fatty acids are transformed into hydroxy fatty acids by gut bacteria such as , which also encodes the enzymes that saturate polyunsaturated fatty acids122.
Polyamines
Polyamines are polycationic compounds with over two amino groups that are biosynthesized from ornithine and arginine. Polyamines, primarily putrescine, spermidine, and spermine, are abundant in the digestive system and are derived from food or biosynthesized by the host and bacteria. Polyamines are chiefly produced within the host by arginase 1 (which converts l-arginine to l-ornithine), ornithine decarboxylase (ODC), which metabolizes ornithine to putrescine, and enzymes that catalysis spermidine, putrescine, and spermine interconversion. In contrary to host polyamine metabolism, bacteria create polyamines using constitutive or inducible amino acid decarboxylases. Polyamines play an important role in cell proliferation, immune system activation, and cell differentiation. Polyamines are crucial during the cell development; a low content of polyamines inside the cell has been associated with cell growth abnormalities. Increased amounts of polyamines are needed by tumor cells relative to normal cells to sustain fast development; increased polyamine concentrations are observed in blood/urine samples from cancer patients relative to those in samples from healthy and normal individuals. Dysregulation of polyamine metabolism in the host or gut bacteria potentially contributes to colorectal cancer (CRC)123. Polyamines in the gut includes cadaverine, putrescine, and spermidine, although bacteria can produce other polyamines. Polyamine pathway enzymes have been found in various organisms. However, only a few species have been functionally characterized in terms of polyamine production. Polyamines are produced, accumulated, or required/used by , and. Cadaverine is a lysine decarboxylation product produced by the bacterium enzymes LdcC and CadA. Cadaverine can be produced by humans and microorganisms. Cadaverine biosynthetic enzymes are also found in and . Polyamine metabolism is dysregulated in pancreatic adenocarcinoma. The fact that modification of the polyamine cycle can amplify or alleviate the effects of traditional cytostatic therapy emphasizes the functional importance of polyamine production in (human) pancreatic cancer124.
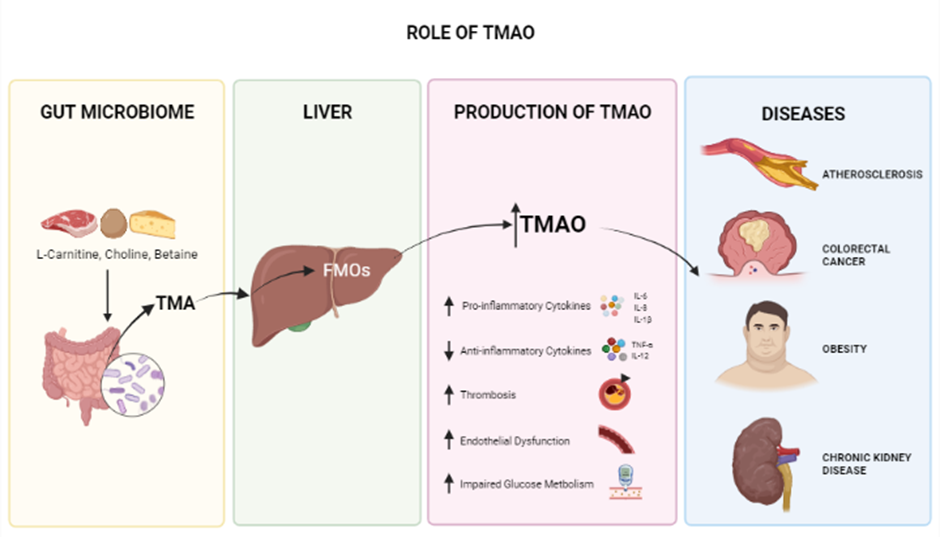
Role of TMAO. TMAO derived from the microbiome has a major role in controlling and regulating major blood and cardiovascular conditions. Increased levels of TMAO have negative effects including inflammation, increased risks of thrombosis, and disturbed glucose metabolism which leads to worsened health conditions; obesity, atherosclerosis CRC and CKD.
Tri-methylamine N-Oxide
Trimethylamine N-Oxide is an osmolyte and a metabolite produced in the gut microbiome and is generated from dietary choline, betaine, and L-carnitine125, 126 crucial for stabilizing protein structures in the presence of urea126. Several studies have shown that high circulating blood levels of TMAO are associated with the development of many chronic diseases, including cardiovascular diseases (CVD), atherosclerosis127, obesity (potentially)128, diabetes, colorectal cancer129, acute kidney disease (AKD) (Figure 2)130, and neurological disorders involving synaptic plasticity131, 132. Since TMAO plays a significant role in heart failure (HF) pathogenesis, one of several cardiovascular diseases (CVDs) caused by vascular inflammation, it can serve as an early and timely prognostic biomarker before HF becomes critical81, 133, 134. This inflammatory response associated with TMAO is also seen in conditions such as psoriatic arthritis (PsA). In a study conducted on 38 PsA patients, TMAO and other chemicals, such as Trimethylamine (TMA), choline, and carnitine, were found to be involved in inflammation. Thus targeting TMAO is a potential treatment for PsA135. In another study of vascular smooth muscle cells (VSMC) from rats and humans, TMAO was found to play a substantial role in the development of inflammatory responses; the Nox4-PRMT5-VCAM-1 pathway was revealed as a potential target for resolving this condition136. Recently, a study of 48 patients with preeclampsia (PE) revealed, by analyzing fecal matter plasma lipopolysaccharide (LPS) and Trimethylamine N-Oxide (TMAO) and in comparison with healthy controls, that elevated LPS and TMAO are present in PE patients137.
Fatty Acids
Short-chain fatty acids are the fermentation products of some gut-microbiome bacteria produced from non-digestible carbohydrates (NDCs)138. The most common SCFA-producing bacteria species are , , and 139. Therapeutically, SCFAs are important because have substantial anti-obesity, anti-inflammatory, and anti-diabetic potential140. Numerous SCFAs, such as valproic acid, acetate, butyrate, and propionate, hold immense therapeutic potential. Valproic acid (VPA) is an anti-convulsant and anti-epileptic SCFA with applications in many neurological disorders, including autism, epilepsy, migraine, and bipolar disorder141, 142. VPA also has anticancer activities1433 μM in combination with arsenic trioxide (ATO) (3 μM showed synergistic anticancer effects in NCI-H460 and NCI-H1299 lung cancer cells and 141. VPA has also shown antioxidant, anti-inflammatory, and antilipidemic properties in a female mouse model with type 1 diabetes. Wistar Rats with type 2 diabetes have also been treated with VPA (100, 300, and 600 mg/kg body weight) and metformin (100 mg/kg body weight), revealing anti-diabetic and pro-antioxidant effects144. Butyrate, another very important SCFA metabolite, is synthesized by gut microbiota and recognized as a very critical mediator in the regulation of whole-body energy metabolism145, particularly functioning as an important nutrient source for colonocytes, influencing their differentiation and growth146. Decreased butyrate levels have been observed in patients with ulcerative colitis (UC), leading to inflammation in the intestinal mucosa. Later it was discovered that butyrate downregulates the expression of genes involved in the inflammatory pathways147
Long chain fatty acids (LCFA) are also therapeutically important. Omega-3 fatty acid (O3FA) is an important long-chain fatty acid not synthesized by the body. It is of great therapeutic interest due to its anti-inflammatory148, antineoplastic149, antithrombotic, insulin resistance, antiarrhythmic150, neuroprotective151, and immunomodulatory152 effects. Eicosatetraenoic acid (EPA) is one of the two main types of O3FA. It has the potential to control the signs and symptoms of depression. Previously, the brain was thought to contain very little or no EPA; however, EPA was recently found to penetrate the blood-brain barrier, where it is immediately esterified into phospholipids; thus, EPA does not build up in the brain and is instead found in at low levels in microglia. Randomized clinical trials are required to demonstrate the therapeutic potential of EPA in combatting major depression153. Recent studies have also demonstrated that EPA is essential for normal brain function. In controlled trials conducted on 92 children (aged 6–12) with attention deficit hyperactivity disorder (ADHD), in comparison with the placebo, high doses of EPA (1.2 g) improved overall focused attention and vigilance. Thus, EPA treatment may improve cognitive symptoms in ADHD-affected youth154. EPA has also shown anti-inflammatory effects155.
Conjugated linoleic acid (CLA) is another naturally occurring LCFA with substantial therapeutic potential due to its anti-carcinogenic, anti-atherosclerosis, and anti-obesogenic properties156. The therapeutic potential of CLA in cancer has been demonstrated in several animal and cellular models studies; clinical trials of CLA for breast cancer and prostate cancer have also been performed157.
4-Ethylephenylesulfate (4-EPS)
4-Ethylephenylesulfate (4-EPS) is a gut-microbiome-derived metabolite considered a uremic toxin158, 159. Elevated levels of 4-EPS have been observed during the atypical neurodevelopment of neuronal tissues in mice. Similarly, 4-EPS entered the brain in another mouse study and induced unusual brain activity and behaviors. In culture, this disturbance of brain activity and behavior patterns was due to the decreased interaction between neuronal cells and oligodendrocytes due to impaired maturation. This impaired activity in mice could be cured by the pharmacological differentiation of oligodendrocytes, demonstrating the toxic effects of 4-EPS on behavior. These gut–brain interactions due to metabolites can be a potential target in treating complex behavior diseases including, autism spectrum disorder (ASD)76, 159. Similarly, higher levels of 4-EPS have been reported in the maternal immune activation (MIA) ASD mouse model, and this increased level of 4-EPS was controlled by the administration of . Axial Biotherapeutics also observed similar elevated concentrations in children with ASD160.
Conclusion
Numerous studies demonstrate the broad potential of microbial metabolites in the treatment of multiple diseases, especially those for which conventional therapies have failed; thus, these metabolites may represent the future of the drug and pharmaceutical industries. The broader efficacy of microbial metabolite therapies remains debatable, and more studies and clinical trials are required. However, previous small-scale studies indicate these therapies are potentially highly effective. Further investigation into microbial metabolites will allow their use to become more common beyond clinical trials. The resulting treatments will likely be comfortably incorporated into approaches for various diseases.
Abbreviations
AD: Alzheimer's Disease, ADHD: Attention Deficit Hyperactivity Disorder, ADI: Arginine Deiminase I, AKD: Acute Kidney Disease, AMPs: Antimicrobial Peptides, ASD: Autism Spectrum Disorder, ASSI: Arginosuccinate Synthetase, ATO: Arsenic Trioxide, CIA: Collagen-Induced Arthritis, CRC: Colorectal Cancer, CSO: Collagenase Santyl Ointment, CVD: Cardiovascular Diseases, EPA: Eicosatetraenoic Acid, EPS: Ethylephenylesulfate, FXR: Farnesoid X Receptor, GIT: Gastro-Intestinal Tract, GST: Glutathione S-Transferase, HF: Heart Failure, HNSCC: Head And Neck Squamous Cell Carcinoma, LAB: Lactic Acid Bacteria, LCFA: Long Chain Fatty Acids, LPS: Lipopolysaccharide, MIA: Maternal Immune Activation, MRSA: Methicillin Resistant Streptococcus Aureus, NAFLD: Non-Alcoholic Fatty Liver Disease, NDC: Non-Digestible Carbohydrates, NSAID: Non-Steroidal Anti-Inflammatory Drugs, ODC: Ornithine Decarboxylase, Pca: Prostate Cancer, PE: Preeclampsia, Pis: Proteosome Inhibitors, PXR: Pregnane X Receptors, SCFA: Short-Chain Fatty Acids, STC: Synaptic Tagging and Capture, TB: TuberculosisTMAO: Trimethylamine N-Oxide, TRD: Taurolidine, UC: Ulcerative Colitis, UPS: Ubiquinone-Proteosome System, VPA: Valproic Acid, VSMC: Vascular Smooth Muscle Cells, WHO: World Health Organization
Acknowledgments
The authors are thankful to the Vice Chancellor, University of Narowal, Narowal, Pakistan for providing the support for the accomplishment of this manuscript.
Author’s contributions
Muhammad Babar Khawar, Muddasir Hassan Abbasi, And Nadeem Sheikh contributed to the study conception and design. Literature Search, data collection and visualization were performed by Ali Afzal, Muhammad Abu Talha Safdar Hashmi, Rimsha Naseem, Nayab Shahid, Adil Farooq, Maryam Mukhtar, Muhammad Ahsan Ashraf, and Syeda Eisha Hamid. The first draft of the manuscript was written by Muhammad Abu Talha Safdar Hashmi, Rimsha Naseem, Nayab Shahid, Rabia Mehmood, and Ali Afzal. Sara Shahzaman, Syeda Eisha Hamid, Muhammad Idnan and Ume Habiba revised the final version of the manuscript. All authors read and approved the final manuscript.
Funding
None.
Availability of data and materials
Not applicable.
Ethics approval and consent to participate
Not applicable.
Consent for publication
Not applicable.
Competing interests
The authors declare that they have no competing interests.