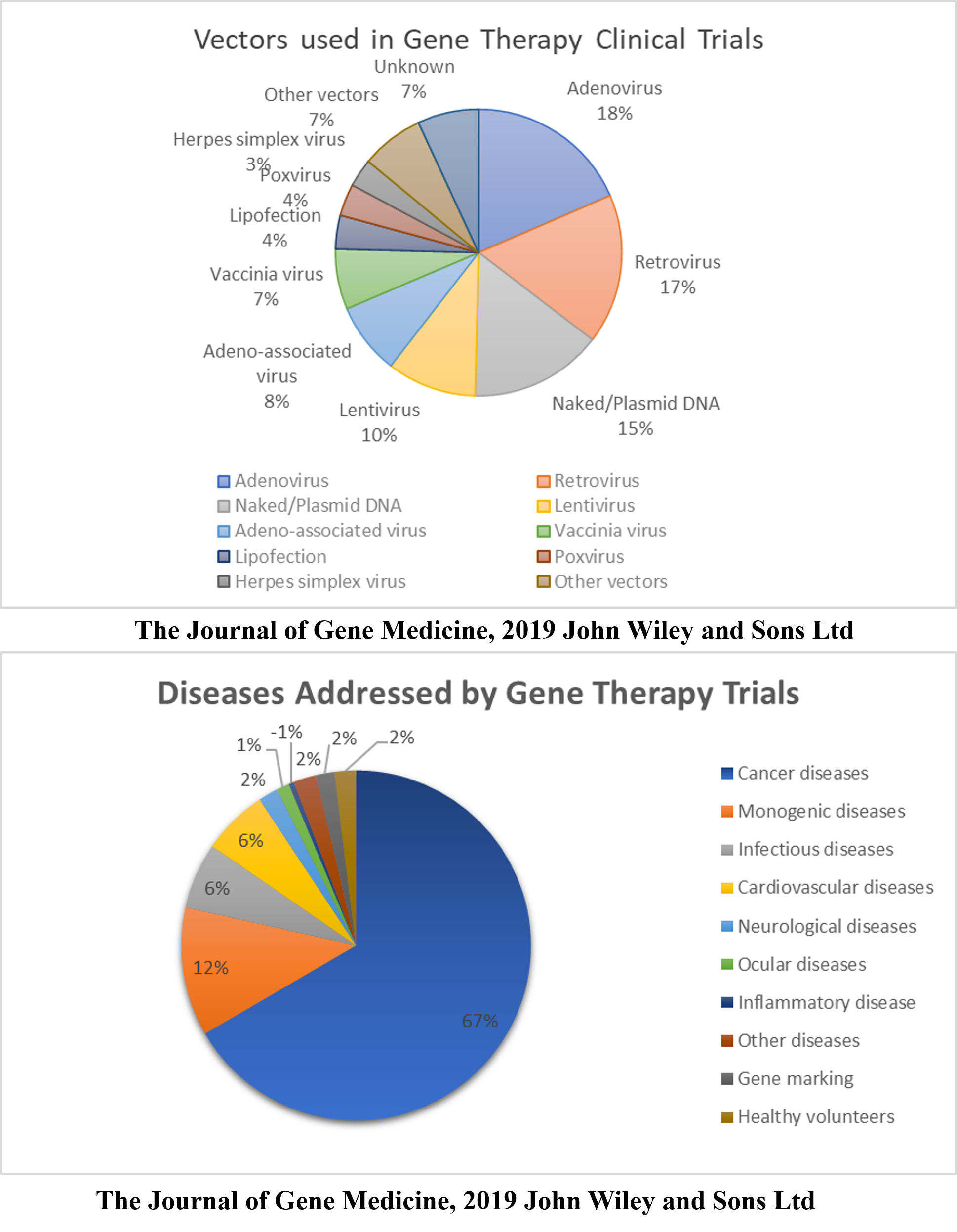
Unlocking effective transgene expression potential in gene therapy with viral vectors
- Lung Stem Cell and Gene Therapy Group, Regenerative Medicine Cluster, Advanced Medical and Dental Institute (IPPT), Universiti Sains Malaysia, Sains@Bertam 13200 Kepala Batas, Penang Malaysia
Abstract
Gene therapy is an experimental approach for treating or preventing disease by using genes. Due to its promised therapeutic benefits for various diseases, this technology has drawn much interest in recent years. Despite reports of adverse events caused by gene therapies, success stories have increasingly emerged. The key to the success of gene therapy is finding a suitable DNA vector that will transport the gene into host cells, thus leading to the expression of the desired protein. An effective vector must be very efficient in delivering a gene to the target cells, non-toxic and safe to patients, inexpensive, and simple to use. This review discusses various aspects of the viral vectors currently in use in gene delivery systems and their great potential to unlock effective transgene expression in gene therapy applications based on this platform.
Introduction
Gene therapy has been defined by the United States (US) Food and Drug Administration (FDA) as a technique that modifies a person’s genes to treat or cure disease (FDA, 2019). On May 22, 1989, Rosenberg and his team attempted the first therapeutic gene transduction using retrovirus-mediated gene transfer, transferring the neo gene into human tumor-infiltrating lymphocytes (TILs). The first-ever approved gene therapy transfer was performed to treat adenosine deaminase severe combined immunodeficiency (ADA-SCID) in 1990. Since then, gene therapy has been regarded as a promising treatment for various inherited or acquired diseases such as cystic fibrosis (CF)1, sickle cell anemia2, heart disease3, cancer4, and acquired immunodeficiency syndrome (AIDS)5. Gene therapy is used to treat or improve the patient’s health condition by delivering exogenous therapeutic genes into the nucleus of target cells. This procedure is performed to treat disorders by correcting the loss-of-function genetic mutation, inhibiting the expression of the disease-causing gene and protein, or expressing the deficient gene product at physiological levels6.
Successful gene therapy involves three critical steps: identifying the faulty gene responsible for the disease, locating the corresponding ‘correcting gene’ or therapeutic gene, and administering gene therapy. A therapeutic gene is a biological product that, when administered into host cells, can regulate genetic sequences and add, delete, replace, or repair genetic sequences. An additional step involves determining a suitable vehicle, known as a vector, to deliver the therapeutic gene into the targeted host cells. Success in gene therapy is largely determined by the effectiveness of the gene delivery method; thus, selecting an appropriate vector is the most essential and difficult aspect of gene therapy. Different viral gene delivery methods and their advantages and drawbacks are described in this study. Clinical trials for human gene and cell-based gene therapy products authorized by the FDA are also included.
Gene Delivery System
An efficient gene delivery system is crucial to ensure successful gene transfer. The ideal gene therapy vector should efficiently target the right cells and effectively deliver the therapeutic gene to the targeted site, such as the cytoplasm or nucleus of host cells. The vector should also demonstrate a dose-response relationship and have a low-risk profile in clinical trials. Several clinical trials of viral vectors failed to achieve optimal therapeutic outcomes. The reasons discussed include poor rates of delivery to the target cells7, and subsequently lower efficacy at the target site, as well as inconsistent transduction efficiency8, 9. The human body is naturally equipped with a sophisticated defense system; hence, circumventing the immune response to the vector is one of the major challenges faced by the gene delivery system. A delivery system must first overcome extracellular barriers (., the delivery system must protect the DNA from degradation, resist deactivation by other extracellular components, and target specific cells or tissues) and then deal with cellular barriers (., the delivery system must promote cellular uptake, escape endosomes, and facilitate the nuclear entry and release of the DNA). In general, gene delivery systems are classified into two groups: viral and non-viral vectors.
Different Groups of Viral Vectors with Their Descriptions, Limitations and Advantages(Adapted from
Vector |
Genetic material |
Packaging capacity |
Tropism |
Inflammatory potential |
Vector genome forms |
Main limitations |
Main advantages |
---|---|---|---|---|---|---|---|
Enveloped | |||||||
Retrovirus |
RNA |
8 kb |
Dividing cells only |
Low |
Integrated |
Only transduces dividing cells: integration might induce oncogenesis in some applications |
Persistent gene transfer in dividing cells |
Lentivirus |
RNA |
8 kb |
Broad |
Low |
Integrated |
Integration might induce oncogenesis in some applications |
Persistent gene transfer in most tissues |
HSV-1 |
dsDNA |
40 kb* 150 kb‡ |
Strong for neurons |
High |
Episomal |
Inflammatory; transient transgene expression in cells other than neurons |
Large packaging capacity; strong tropism for neurons |
Non-enveloped | |||||||
AAV |
ssDNA |
<5 kb |
Broad with the possible exception of hematopoietic cells |
Low |
Episomal (>90%) Integrated (<10%) |
Small packaging capacity |
Non-inflammatory; non-pathogenic |
Adenovirus |
dsDNA |
8 kb* 30 kb§ |
Broad |
High |
Episomal |
Capsids mediate potent inflammatory reactions |
Extremely efficient transduction of most tissues |
Viral vectors include various viral species with different nucleic acid compositions and characteristic features related to the host cell specificity, expression pattern and duration, and cytotoxicity (
Viral Vectors
Since the onset of the gene therapy era, viral vectors have been used as a primary vehicle for gene delivery to human cells. In 2019, over 3000 clinical trials related to gene therapy applications were conducted, proving that this field is gaining significant interest14. The clinical trials focused on cancer therapy, monogenic diseases, infectious diseases, and cardiovascular diseases. Interestingly, although more efficient non-viral vector-based methods have been developed recently, viral vectors were used in nearly 70% of the trials.
The recombinant viral vectors often used in gene therapy applications include Ad, RV, lentivirus (LV), HSV, and AAV, which range in their genomic size from ~5 to 150 kb. The suitability of each type of viral vector for different applications is decided based on various features. There are two major classes of recombinant viral vectors generally used to deliver genes, grouped according to whether their genomes exist as extrachromosomal episomes (AAVs, HSVs, and Ads) or integrate into the recipient’s chromosome (RVs and LVs). This feature is crucial for determining the suitability of each vector for certain applications. Although many targeted diseases have been responsive to gene therapy, no single vector is suitable for use in all applications. The essential features required of all vectors include assured safety, reproducibility, stability of propagation, the ability to be purified to high titers, and the ability to mediate specific targeted gene delivery, where only the therapeutic gene specific to the tissue or organ of interest is transferred without widespread vector dissemination. The features of these five commonly used viral vectors are discussed in detail in the following section.
Adenoviral vectors
Ads are non-enveloped viruses with a double-stranded DNA genome and icosahedral nucleocapsid. Around 57 previously discovered human Ad serotypes are divided into 7 subgroups (A–G)15. The most extensively characterized Ads are the 36-kb serotypes 2 (Ad2) and 5 (Ad5), which belong to subgroup C. These Ads, especially those of serotype 5, are widely used in a broad range of gene therapies because they can transduce various types of dividing and non-dividing cells both and 16. Most recombinant adenoviral systems designed for gene transfer applications are replication-defective or, in other words, not capable of replicating themselves15, 17, 18. They are constructed into a vector expressing the therapeutic gene, while a helper construct encodes for the viral proteins and makes the virus incapable of replicating19. Recombinant Ad vectors are the most efficacious medium by which to deliver genes . In 2013, 476 human gene therapy studies were conducted using recombinant Ad vectors, representing 23.5% of all gene therapy trials; with this, Ad vectors were recognized as the viral vector most applied in clinical trials globally20. This is due to their broad cellular tropism for and applications. Despite having significant advantages for various gene therapy applications, Ads lack native integration machinery that would enable the attainment of permanent gene expression . Ad vectors do not integrate into the host genome and remain episomal. Studies have demonstrated that random integration of replication-defective Ad vectors into the host genome occurred at frequencies of 0.001% – 1% of infected cells, reducing the risk of insertional mutagenesis. Current advances have led to the emergence of a range of hybrid vectors combining the vastly efficient DNA delivery of Ads with the integration machinery of RVs, AAVs, and transposons to improve the integration frequencies of Ad vectors. These hybrid vectors have shown promise, at least in systems21.
Ad vectors are highly immunogenic and can induce strong innate and adaptive immune responses. Recombinant Ad vectors have been constructed by deleting numerous parts of their coding regions. The first-generation Ad vectors were made by substituting the early gene (E1) with a transgene. Although the deletion of E1 resulted in a replication-defective vector, the E2, E3, and E4 promoters remained active and led to the expression of viral genes at low levels15. This leaky viral protein expression induced strong cytotoxic immune reactions that rapidly eliminated transgene expression in transduced cells19. Additional attempts were made to delete several early genes (E2a, E2b, or E4) besides E1 to reduce the immune response to the viral antigens produced due to leaky expression. Although these second-and third-generation Ad vectors reduced toxicity, the gene sequences encoding the viral particles remained active, leading to the expression of viral proteins that induced secondary innate and adaptive immune responses against the vectors22, 23. Because of these factors, the sufficient expression period for Ad vectors in humans peaks at 1 week and is restricted to about 2 weeks, making these vectors ideal for use whenever a shorter duration of vector expression is required, such as for generating new biological structures20. Despite the safety of Ad vectors, considering the immune response against them, helper-dependent Ad (HDAd) vectors with large-scale cloning capacity were introduced. All viral genes except the inverted terminal repeats and packaging signal are deleted in these HDAd vectors24. HDAd vectors were found to reduce chronic toxicity in comparison to the first- and second-generation viruses in which only a part of the viral genome was deleted25. Although the safety profiles of HDAd vectors were improved, the dose of the vector was shown to trigger an acute immune response mediated by capsid proteins of the vector in a dose-dependent manner26.
To sum up, Ad has a high transduction capacity and is the most effective gene delivery method in cell and tissue types of varying complexity. The strong inflammatory response to the virus’s capsid proteins is the greatest flaw of the Ad system. It is anticipated that additional development will soon provide patients with efficient and safe Ad gene transfer.
Retroviral vectors
RVs, which are enveloped viruses with RNA genomes, can reverse transcribe their genetic material from RNA to cDNA. Most recombinant RV systems are replication-defective, and a transgene expression vector along with a helper virus that expresses viral proteins are used in gene therapy applications. However, the ability of the virus to replicate is limited. Packaging features are provided by the helper virus that carries co-transfected plasmids in a packaging cell (., the HEK293 cell line). The packaging cell lines encode sequences involved in viral packaging functions (gag, pro, pol, and env). These genes cannot be packaged into RV particles because they are supplied during transformation using molecular constructs. The transgene is delivered into a genetic vector that mimics the viral genome structure but contains fewer cis-acting sequences, permitting its effective incorporation into viral particles, reverse transcription, and expression in target cells.
The use of RV vectors is a classic approach in long-term gene therapy applications, and RVs have been used to deliver transgenes for the past three decades9. As the gold standard, RV vectors could accommodate up to 8 kb of foreign genes and were proven effective in therapies involving bone marrow stem cell transplants27. The most commonly used RV vector is derived from the murine leukemia virus (MLV). In 1990, the first human gene therapy using MLV-based gamma-retroviral (γ-RV) vectors carrying therapeutic genes was performed in two young girls suffering from severe combined immunodeficiency (SCID). The clinical trial involved the implantation of autologous bone marrow cells transduced with gamma RV vectors28, 29. Although the clinical trial restored T-cell immunity in the SCID patients, vector-induced insertional oncogenesis due to random incorporation of the vector into the host genome was identified as a significant issue30, 31, 32. Another limitation of RVs is their inability to infect non-dividing cells, which enhanced the interest in applying LV vectors for gene therapy. LVs are capable of infecting both dividing and non-dividing cells even though they belong to the RV family. Therefore, LVs exhibit low cytotoxicity33.
In sum, because of their persistent and long-term gene transfer in proliferating cells, RVs are a promising option for gene transfer. However, RVs only transduce diving cells and integration may lead to oncogenesis in certain cases.
Lentiviral vectors
LVs are a type of RVs. Scientists showed interest in LVs for the first time in the early 1980s when the AIDS pandemic introduced the world to the most well-known and feared LV: human immunodeficiency virus type 1 (HIV-1). Since replication-defective LVs derived from HIV-1 can incorporate into the genome of non-dividing cells such as neurons and hematopoietic stem cells (HSCs) with a sustained, long-term expression of therapeutic genes, they were adopted as vehicles for gene delivery33. This is a unique feature of LVs as other RVs require proliferating cells to integrate into the cellular genome34. These properties underscore the potential benefits of using LVs over other RV vectors in human gene therapy applications. Naldini (1996) were the first to show that LVs derived from HIV-1 could offer efficient preclinical gene delivery, stable incorporation into the cellular genome, and constitutive expression of the therapeutic genes in non-dividing cells such as neurons35. However, potent host immune responses against the LV capsid and/or their transgene products hinder the success of gene therapy using LV vectors. The transgene expression period and potency of immune reactions to LV-encoded transgene products have differed among studies on delivery. The efficacy of LV vector-mediated gene delivery in immunocompetent mice leads to effective transduction and transgene expression in various cell types, including the retina, muscle, and hematopoietic cells, but the transduction of hepatocytes is relatively inefficient.
LVs function like RVs in that the genomic RNA of LVs is reverse-transcribed into cDNA as the virus invades the cells. The cDNA produced is then integrated into the cellular genome with the aid of a viral integrase enzyme at a random site. The integrated viral genome, now recognized as a provirus, remains in the host genome and is transferred to cell progeny as the cells undergo division. However, the integration site cannot be predicted, which may lead to some issues. The function of cellular genes may be interrupted by the provirus, inducing oncogene activation and enhancing carcinogenesis, raising safety concerns around the use of LVs in gene therapy.
Nevertheless, a few studies showed that the selection of LV insertion sites differs from that of RVs such as γ-RV vectors. This could be due to the differences in the structure of the integrase enzyme of these two types of viruses and the viral long terminal repeats (LTRs) in the γ-RV vectors. LVs incorporate randomly throughout the genome, although they only target active transcription units. On the other hand, the integrase and LTRs of γ-RV vectors were found to promote selected interaction with active host cell promoters and enhancers36. A previous study conducted by Aiuti et al. suggested that lentiviral gene therapy is safer than retroviral gene therapy since LVs have a lower risk of causing insertional mutagenesis. This lentiviral gene therapy did not promote the selection of integration sites near oncogenes and there was no aberrant clonal expansion detected37, 38.
The use of lentiviral vectors in gene therapy has emerged owing to the persistence of gene transfer in most tissues after the insertion of the vector. Its biggest flaw is integration possibly leading to oncogenesis in certain situations.
Herpes simplex virus vector
HSVs are large, enveloped viruses belonging to the subfamily of the family . Amongst the herpesviruses, HSV serotype 1 (HSV-1) has several natural properties that make it attractive for use as a viral vector, particularly for treatments related to diseases of the nervous system12, 39. In contrast to other viral systems, HSV-1-derived vectors can package and efficiently transfer up to 150 kb of transgenes to the nucleus of mammalian cells. HSV-1-based vectors can accommodate multiple transgene cassettes or single large genes, which is very practical for applications that require 97 expressions of one or a few gene products for an effective therapeutic outcome (., tumor-killing). HSV-1-based vectors can infect many cell types, including both dividing and non-dividing cells; however, they cannot incorporate into cellular DNA40, 41. These viruses lack lytic viral protein production and thus are episomal. Interestingly, latent, lifelong infections in the nervous system with the long-term expression of the transgene in brain neurons and without any leaky expression of the viral genes make HSV vectors a promising vehicle for gene delivery to the central nervous system (CNS)42. Several clinical studies using HSV vectors were performed to test therapies against recurrent breast cancer, head and neck cancer, unresectable pancreatic cancer, refractory superficial cancer, melanoma, and other conditions, mainly neurological and pain disorders43.
Amplicons are vectors harboring only a minimal number of virally derived sequences and are non-integrative, replication-defective, and helper-dependent HSV-1-derived vectors. They have the same properties as typical HSV-1 particles, including structure, tropism, and immunogenicity. However, they carry a concatemeric DNA plasmid containing the HSV-1 origin of replication (ori), HSV-1 packaging signal (pac), and transgene rather than a functional HSV genome44. These sequences enable the packaging of infectious HSV-1 particles. HSV-1 amplicon vector systems offer some advantages in gene therapy. They do not carry any viral genes, providing more space to accommodate larger foreign DNA molecules (up to almost 150 kb). These advantages make the HSV vector a powerful, interesting, and useful gene delivery tool.
Amplicons are completely safe for inoculation in animals, non-toxic to the infected cells, and only induce low levels of the adaptive immune response because they do not encode any viral proteins45. Like other HSV vectors, amplicons remain episomal, therefore posing no risk of insertional mutagenesis. Moreover, HSV-1 naturally spreads in the nervous system via neuronal transport, and it infects several species, which is useful for the assessment of vectors in various animal models. Transduction in the brain is generally more effective with HSV vectors than with other vectors (lower titers are required) since neurons are the natural target for HSV-1 infection.
To summarize, the benefits of HSV-1 vectors include their ability to infect both quiescent and proliferating cells effectively as well as their ability to encode for transgenes of significant size. On the other hand, when primary fibroblasts are used, there is a rapid reduction in the expression of the transgene.
Adeno-associated virus vector
AAV, a small, non-enveloped virus that infects human cells, is naturally replication-defective and does not cause disease in humans. So far, AAV appears to be the most promising means of gene delivery since the wild-type virus has never been reported to cause any disease in humans46, 47. The general principles of recombinant AAV (rAAV) vector construction involve the modification of molecular clones by replacing the AAV coding gene sequence with exogenous DNA to generate a plasmid vector. Recombinant vectors derived from these viruses are composed of two inverted terminal repeats flanking an expression cassette encoding a therapeutic transgene, deleting all viral open reading frames. To propagate rAAVs, co-infection with a helper virus, mainly Ad, is required47. This system facilitates rAAV production at relatively high titers. However, this preparation is contaminated with the helper virus and therefore needs further purification. For gene therapy applications in humans, this requirement for co-infection offers a natural safety feature that helps prevent the improper spread of rAAVs following clinical administration.
AAVs have a good safety profile for use as a vector in gene therapy. Because of the absence of viral genes, AAVs do not cause a deleterious host immune response47. A clinical trial in hemophilia B patients using an AAV-2 vector resulted in long-term expression in skeletal muscles and deficient inflammatory responses48, 49. However, the effectiveness of AAVs relies on the serotype, administration route, effective multiplicity of infection of the vector employed, transgene and expression cassette, and dosing schedule of injection50. Although AAV vectors have low immunogenicity, a primary host immune response (., a neutralizing antibody response) may be elicited against the vector capsid protein or transgene product, which might impact their use. For example, the decline in expression of the factor IX transgene 6 weeks after hepatic gene transfer and destruction of hepatocytes have been observed in patients in the highest dose cohort. Other significant positive features of AAV vectors include their efficacy in transducing proliferating cells as well as non-proliferating tissues, such as neurons, photoreceptor cells, hepatocytes, and muscle cells and their promotion of the long-term expression of transgenes . This is due to their integration into the host chromosome. Although AAV vector genomes can persist within cells as episomes, vector integration has been seen in numerous experimental settings51. About 20 AAV vectors were used in clinical settings for various therapeutic and prophylactic applications (., hemophilia B and CF)52. As of 2020, AAV vectors have been used in more than 12 clinical studies on hemophilia alone53.
Although AAV has a good safety profile compared to other viruses and may mediate long-term gene expression in non-proliferating or slowly proliferating cells, it is limited by several factors. For example, the immune response was shown to be triggered by the repeated administration of AAV vectors54. To overcome this problem, a different AAV serotype is applied for each re-administration. Another related issue is the very small packaging size of AAV (~4.7 kb), which limits the size of transgenes that can be packaged into the vector55. AAV vectors are unable to efficiently infect certain types of cells, such as HSCs, which are considered potential targets for numerous gene therapy applications. Furthermore, the safety of AAVs as a vector in clinical applications is limited because of concerns about AAV integration-mediated tumorigenicity. A previous study reported that the development of hepatocellular carcinoma was associated with AAV gene delivery in mice56.
AAVs offer similar benefits to Adv vectors, such as high transduction efficiency and long-lasting transgenic expression. They have a high biosafety rating and low immunogenicity, making them a good choice for gene therapy. However, AAVs also have several drawbacks, including a reduction in transgene expression over time due to episomal integration and a modest packaging capacity.
Administration Routes and Their Importance
Gene therapy can be performed either or , and the modality is decided based on other factors such as the nature of the cell, required expression levels, and safety. The technique involves directly injecting the target gene into patients, while the method involves isolating and transfecting cells before reinjecting them into patients. Selecting administration routes for targeted gene delivery is crucial to determine the fate of the administered viral vector. Administration routes may be divided into two categories: systemic routes and local (topical) routes. The method of administration used to deliver the transgene is one of the variables that influence its expression. Oral, intravenous (IV), and topical administration routes are all options for viral vectors.
Oral administration
Oral gene therapy remains a promising and attractive strategy despite its challenges and obstacles. Oral administration holds great promise as it allows the daily intake of genetic medicine, easing the administration route and allowing transient gene expression. The idea of oral gene therapy is exciting because the body is not subjected to invasive surgery or deep wounds or exposed to local drug effects upon administration. Gene therapy clinical trials using oral administration that are actively recruiting participants include those testing treatments for typical Canavan disease in children57 and high-risk prostate cancer58 using AAV and Ad, respectively. The main obstacles to effective oral administration of gene therapy include withstanding harsh and everchanging conditions, such as the acidic pH and degradative enzymes in the gastrointestinal tract, without compromising the transgene while simultaneously maintaining efficiency.
IV administration
IV administration, also known as systemic delivery, is one of the most preferred viral gene delivery methods. IV administration is well tolerated at higher viral doses and provides a high level of gene expression. It has been reported as an effective and safe route for cancer gene therapy using HSV59. One of the system’s limitations is its broad distribution of vectors throughout the body that can easily lead to side effects. For instance, liver tropism is often observed after Ad administration through the IV route.
Topical administration
One of the major challenges in gene delivery is to deliver the transgene specifically to the target tissue; in this regard, local gene delivery holds great potential compared to systemic gene delivery. In the last 5 years, 40% of ongoing Phase III clinical trials on cancer gene therapy have utilized local gene delivery systems. A study on bevacizumab delivery using an AAV gene system showed sustained gene expression with less off-target effects and toxicity in lung cancer60. Another study showed that regional IV application using suitable AAV vectors has the most promise as a gene therapy approach for ischemic heath disease61. Local delivery, like pleural delivery, was also shown to have little risk of gene- or virus-induced inflammation62.
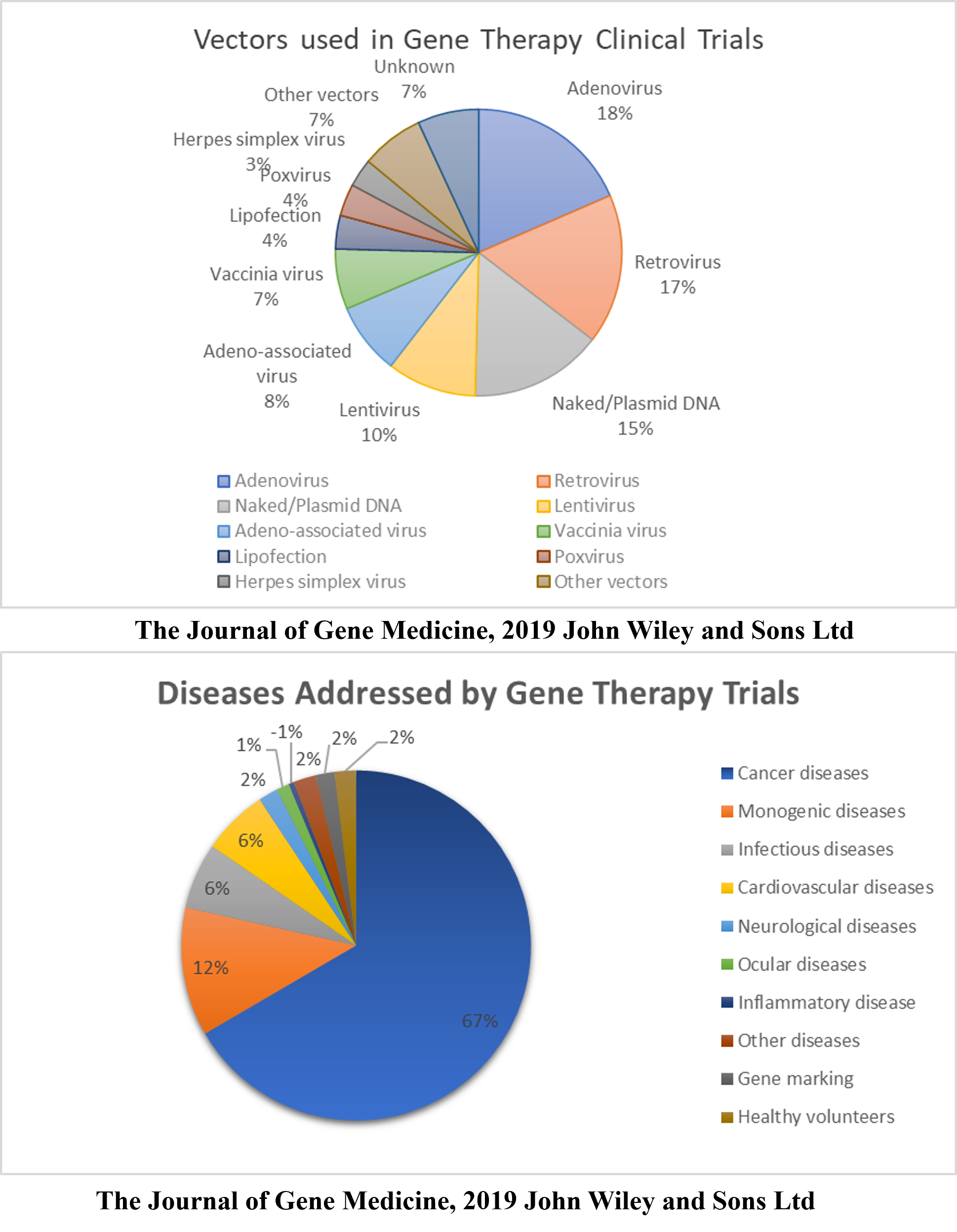
Percentage of gene therapy trials devoted to different patient groups. Initial clinical trials focused on monogenetic diseases. However, cancer has become a major interest in recent years. https://doi.org/10.6084/m9.figshare.16752061.v1
Gene Therapy Clinical Trials
According to a database provided by the Journal of Gene Medicine in their Gene Therapy Clinical Trials worldwide site, 3143 gene therapy studies have been performed globally thus far (http://www.wiley.co.uk/genmed/clinical, updated on Sept 2019). Initial clinical trials focused on monogenic diseases. However, cancer has become a major topic of interest in recent years (~67%), followed by monogenic, cardiovascular, infectious, and neurological diseases (Figure 1). Based on the clinical trials performed, virus vectors were found to be more efficient than non-viral vectors. As many as 67% of the clinical trials used viral vectors, including Ads, RVs, AAVs, LVs, vaccinia virus, poxvirus, and HSVs (Figure 1). An updated list of all approved gene therapy products, including viral and non-viral vectors, is given in
List of Approved Gene Therapy Products (adapted from
Trade name |
Approval authority (date) |
Indication |
Manufacturer |
Clinical trial I.D. |
Vector |
---|---|---|---|---|---|
Fomivirsen (vitravene) |
USA FDA (1998) EMA (1999) |
tCMV retinitis |
ISIS Pharmaceuticals with Novartis Ophthalmics |
NCT00002187 NCT00002156 NCT00002356 NCT00002355 |
ASO – inhibition of immediate-early IE-2 protein |
Gendicine |
State Food and Drug Administration of China (2003) |
Head and neck squamous cell carcinoma |
Shenzhen SiBiono GeneTech (Shenzhen, China) |
ChiCTR-TRC-08000094 ChiCTR-TRC-08000092 ChiCTR-TRC-08000091 |
Adenovirus |
H101 (Oncorine) |
China FDA (2005) |
Patients with last stage head and neck cancer, lung cancer, liver cancer, malignant pleural and peritoneal effusion and pancreatic cancer |
Shanghai Sunway Biotech |
NCT02579564 |
Adenovirus |
Rexin-G |
Philippine FDA (2007) USA FDA (2010) |
A solid metastatic tumour (pancreatic, breast cancer, osteosarcoma, sarcoma |
Epeius biotechnologies |
NCT00505271 NCT00572130 NCT00505713 NCT00504998 NCT00121745 |
Retrovirus |
Neovasculgen (PI-VEGF165) |
Russian ministry of healthcare (2012) |
Atherosclerotic peripheral arterial disease (PAD) |
Human stem cells institute |
NCT03068585 NCT02538705 NCT02369809 |
CMV |
Glybera® (alipogene tiparvovec) |
EMA (2012) |
Lipoprotein lipase deficiency |
uniQure (Amsterdam, Netherlands) |
NCT02904772 NCT03293810 |
AAV1 |
Strimvelis™ |
EMA (2016) |
Adenosine deaminase deficiency (ADA‐SCID) |
GlaxoSmith-Kline (Middlesex, United Kingdom) |
NCT00598481 |
Retrovirus |
Zalmoxis |
EMA (2016) |
Hematopoietic stem cell transplantation graft vs host disease |
MolMed SPAA |
TK007 TK008 |
Retrovirus |
Imlygic |
USA FDA (2016) |
Multiple solid tumors (melanoma, Pancreatic cancer) |
Amgen |
NCT02014441 NCT01740297 NCT02366195 NCT00402025 |
HSV-1 oncolytic virus |
Kymriah™ |
USA FDA (2017) |
Acute lymphoblastic leukaemia |
Novartis Pharmaceuticals (Basel, Switzerland) |
NCT02435849 NCT02445248 |
CART- Lentivirus |
Yescarta™ (axicabtagene ciloleucel) |
USA FDA (2017) |
B‐cell lymphoma |
Kite Pharma, Incorporated (Santa Monica, California, USA) |
NCT02348216 |
CART - Retrovirus |
Luxturna™ (voretigene neparvovec‐rzyl) |
USA FDA (2017) EMA (2018) |
Retinal dystrophy, retinal pigment epithelial cells |
Novartis Inc |
NCT00999609 NCT03602820 |
AAV2 |
INVOSS (TissueGene-C or Tonogenchoncel-L |
South Korea's MFDS (2017) |
Kellgren-Lawrence Grade 3 knee osteoarthritis |
TissueGene (now called Kolon TissueGene) |
NCT01221441 NCT03412864 |
Retrovirus |
Zolgensma |
USA FDA (2019) |
Pediatric individuals less than 2 years of age diagnosed SMA having bi-allelic mutations in the SMN1 gene |
AveXis/Novartis |
NCT03421977 NCT03505099 NCT03421977 NCT02122952 NCT0330627 NCT03461289 |
Recombinant AAV9 |
The earliest study on gene therapy was conducted in 1989 at the National Institute of Health by Rosenberg l. (1990), in which a retroviral vector was used to transfer a neomycin resistance marker gene into TILs from five metastatic melanoma patients65. Adoptive transfer of TILs, previously reported to have unique lytic specificity for autologous tumors, along with interleukin-2, resulted in tumor regression. This successful study proved that transferring genes using RV is practical, leading to many other studies66.
In 2000, the first successful gene-based clinical trial was performed. An RV vector was used to treat 20 infants suffering from SCID-X163. A trial conducted in France on long-term exposure to RV revealed that eight out of nine treated patients were alive after a median period of 9 years. However, four developed leukemia after 30 months of treatment31.
In the context of HSV-based clinical trials, an oncolytic HSV-HF10 vector has been used in various clinical trials on recurrent breast cancer, head and neck cancer, unresectable pancreatic cancer, refractory superficial cancer, and melanoma43. These clinical trials showed high safety and a low frequency of adverse effects in treated patients. In Phase I clinical trials, the oncolytic HSV G207 vector lacking genes essential for replication in normal cells was evaluated in patients with recurrent glioblastoma multiforme67. No patients were found to develop HSV encephalitis, but significant antitumor activity was observed following two doses of HSV G207. In another Phase I clinical trial, six of nine patients had stable disease or partial response. The median survival time was 7.5 months after a single dose of oncolytic HSV was injected into patients with recurrent/progressive high-grade glioma, indicating the potential for clinical response68.
Ads, AAVs, and Sendai virus (SeV) have been acknowledged as effective gene therapy vectors to treat CF. The first CF gene therapy study involving a viral vector was reported in the early 1990s, where Ad serotype 2 was used to transfer the CF transmembrane conductance regulator (CFTR) gene encoding human cDNA to the nasal epithelium of three CF patients69. This study successfully provided proof-of-concept of correcting defects in the chloride transport system using the Ad vector and led to further studies using adenoviral vectors. Human parainfluenza virus (HPIV) and SeV were also shown to efficiently transfer genes into airway epithelial cells70. However, only SeV was demonstrated to partially fix chloride transport defects in CF knockout mice71. Since these viral vectors have a natural tropism for the airway epithelium, they only showed transient expression and were inefficient upon repeated administration. Therefore, LVs that can transduce both proliferating and non-proliferating cells were established as the vector for gene transfer in CF treatment72. In 2015, the United Kingdom CF Gene Therapy Consortium (UK CFGTC) completed the Phase IIb multiple-dose clinical trial of CFTR-expressing plasmid delivery mediated by liposomes. The CFTR-expressing plasmid was administered once a month for 1 year, and this trial was the first to demonstrate that gene therapy may serve the moderate stabilization of lung function in CF patients73.
Apart from CF, the substantial advancement of the LV-based gene therapy approach has led to promising results for a dozen clinical trials for the treatment of genetic diseases. For instance, autologous CD34cells transduced with lentiviral vectors encoding the human βA-T87Q-globin gene administered to 18 β-thalassemia patients carrying mutations in the HBB globin gene in Phase I/II clinical trials proved to be successful in replacing long-term allogeneic hematopoietic cell transplantations74. There was no significant toxicity related to the vector 3 years post-infusion as found in patient follow-ups. This result directed the development of LentiGlobinBB305, which has now advanced to a multinational Phase III clinical trial for the treatment of β-thalassemia (NCT03207009 and NCT02906202) and sickle cell disease (NCT04293185)75.
At least 12 clinical trials using AAV vectors to treat hemophilia, a severe blood-clotting disease, are currently ongoing. The majority of these clinical trials are in the Phase II/III stages and the tested interventions hence exhibit promise for commercialization. For example, fidanacogene elaparvovec, developed to treat hemophilia B; SPK-8011, gene therapy for hemophilia A; Valrox (valoctocogene roxaparvovec or BMN 270), gene therapy for hemophilia A; and AMT-061, gene therapy for hemophilia B, are currently being tested in phase III clinical trials (NCT03392974 and NCT03569891). FLT180a is also being tested in a Phase II/III study on hemophilia B patients in the UK (NCT03641703)75. Apart from hemophilia, a recent Phase I clinical trial to treat age-related macular degeneration (AMD) and diabetic macular degeneration using AAV vectors has shown positive outcomes in terms of disease reversal (NCT03748784). This clinical trial aimed to evaluate the safety and tolerability of a single intravitreal injection of an AAV vector carrying aflibercept (an anti-VEGF fusion protein that blocks blood vessel growth and leakage related to AMD and diabetic retinopathy in patients with AMD), correction of visual acuity, and elimination of anti-VEGF rescue injections. The trial resulted in mean best-corrected visual acuity improvements of +6.8 letters and central subfield macular thickness reduction of −137.8 μM, indicating disease reversal in patients receiving treatments76. This choice of gene therapy is obvious due to the application of the evolved capsid that is strongly tropic to photoreceptors known as AAV2.7m877.
Despite many technological challenges and barriers, numerous gene therapy-based drugs have entered the global pharmaceutical market (
Challenges and Hurdles in Gene Therapy/Risks of Gene Therapy
Although gene therapy has enormous potential, several adverse events due to risks associated with the viral vector have been reported. The first adverse event happened in 1999 at the University of Pennsylvania, where a gene therapy involving Ad administration resulted in the death of 18-year-old Jesse Gelsinger, who suffered from a partial deficiency of ornithine transcarbamylase (OTC). OTC is a liver enzyme that removes excess nitrogen from amino acids and proteins64, 79. After 4 days of treatment, Gelsinger died due to a hyperimmune reaction after a very high dose of Ad caused his organs to stop functioning.
The impressive success of gene therapy clinical trials for SCID-X1 in 2000 gave rise to a new era of therapeutics using RVs as vectors. First, a clinical trial was successfully conducted involving five patients who underwent RV-mediated transfer of the γc gene into CD34 cells. The second trial involved four additional patients, three of whom were doing well up to 3.6 years later, whereas the fourth patient died. However, between 30 and 34 months after gene therapy, the three remaining patients developed T cell leukemia. This was due to proviral integration within the LMO-2 locus located on the short arm of chromosome 11. The proviral integration resulted in aberrant expression of LMO-2, which led to the generation of acute lymphoblastic leukemia from T cells with the α/β receptor. This study highlighted the risk of insertional mutagenesis events directly linked to virus-mediated gene transfer80.
Conclusions
The effectiveness of gene therapy relies heavily on the transfer vector. To date, viral vectors are the best available vehicles due to their relatively high delivery efficiency to most tissues and have been the main vectors used in gene therapy clinical trials. Certain viruses are frequently employed as vectors since they can transport the new gene by infecting the cell and have been engineered not to cause harm to patients. The viral vector’s capacity to integrate into the host genome, such as in the case of RVs, results in permanent and persistent gene expression. Although some drawbacks of using viral vectors have been reported, such as random insertion into the host genome that could potentially cause oncogene activation, the limited insertion capacity of target genes, low titers, and inability to transduce non-dividing cells, these drawbacks do not prevent them from unlocking their effective transgene expression potential in gene therapy applications.
Abbreviations
AAV: Adeno-associated virus
Ad: Adenovirus
ADA-SCID: Adenosine deaminase severe combined immunodeficiency
AIDS: Acquired immunodeficiency syndrome
C.F.: Cystic fibrosis
CFTR: Cystic fibrosis transmembrane conductance regulator
CRISPR: Cluster regularly interspaced short palindromic repeats
HDAdVs: Helper-dependent Ad vectors
HPIV: Human parainfluenza virus
HSV: Herpes simplex virus
LTRs: Long terminal repeats
LV: Lentivirus
MIDGE: Minimalistic, immunological-defined gene expression
MLV: Murine leukaemia virus
OTC: Ornithine transcarbamylase
rAAV: Recombinant AAV
SCID: Severe combined immunodeficiency
SeV: Sendai virus
siRNA: Small interfering RNA sequences
Acknowledgments
We thank all the authors who have made substantive contributions to this article.
Author’s contributions
Nur Shuhaidatul Sarmiza and Noorhanis Abu Halim contributed to the conception and design of the article. Nur Shuhaidatul Sarmiza, Noorhanis Abu Halim, Norashikin Zakaria and Lelamekala participated in drafting the article. Ida Shazrina was involved in the critical revision of the article. Badrul Hisham Yahaya was involved in the review, comments and approved the version to be published.
Funding
This work was supported by Fundamental Research Grant Scheme (FRGS), Ministry of Higher Education Malaysia (203/CIPPT/6711509 and 203/CIPPT/6711725) and Research University Grant Scheme Universiti Sains Malaysia (1001/CIPPT/8012203).
Availability of data and materials
Not applicable.
Ethics approval and consent to participate
Not applicable.
Consent for publication
Not applicable.
Competing interests
The authors declare that they have no competing interests.